Asia Pacific J Clin Nutr (1995) 4: 95-101
The interplay between nutrition
and body composition
P Fürst1 and H Leweling2
- Department of Biological Chemistry
and Nutrition, University of Hohenheim, Stuttgart, Germany
- Medical Clinic Mannheim, Department
of Pathophysiology, University of Heidelberg, Germany
Stress and malnutrition are associated with altered
body composition. Extracellular fluid increases, with wt gain,
but in response to stress BCM may gradually shrink with wt loss.
In catabolic illness there is extracellular fluid expansion and
erosion of AT and BCM. In stress, net loss of body fat was associated
with interstitial accumulation of lipids preferentially in muscle,
although BIA did not indicate increased fat and decreased water.
Severe trauma and sepsis exerted prolonged effects on tissue electrolyte
and water metabolism. Treatment of the critically-ill is of the
primary illness. Nutritional therapy is an effective adjunct except
in chronic sepsis or critical patients with MOF with great wt
and protein loss. Glutamine dipeptides may help with cellular
hydration and address catabolic changes.
Introduction
Injury, sepsis, malnutrition as well as dietary intake
all have important effects on body composition and therefore, on the
therapeutic approaches to be adopted. Indirectly these factors may
also influence the goals of nutritional therapy.
Rapid weight loss, due to loss of body fat and skeletal
muscle mass, frequently accompanies short-term self-limiting disease
processes like injury and infection1,2. Similar catabolic
events are associated with other disorders, like diabetic ketoacidosis,
multiple-organ failure, chemotherapy or radiation treatment for cancer3,4.
The loss of body tissue may be minimal and of little consequence in
a patient with normal nutritional status and a brief uncomplicated
illness. Severe complications are however to be expected during prolonged
illness in nutritionally-depleted patients. In the long-term, these
complications prolong convalescence and impede recovery2.
For a proper understanding of the alterations brought
about by nutritional and metabolic imbalances during illness and recovery,
detailed information on the morphological changes induced, and on
their accompanying physiological and biochemical effects, is required5.
Recent technological advances, like nuclear medicine, radiology and
medical physics have opened up new possibilities for measurement of
body composition. However, there are considerable limitations in applying
such measurements to clinical situations. Indeed, such methods are
as yet confined to research centres and hospitals and their progression
further into the community seems at present unlikely6.
The need for simple and valid techniques is thus a growing concern
of practitioners in clinical nutrition.
Irrespective of the considerable limitations of the
use of these methods, studies devoted to body composition have contributed
much valuable information and gained new impetus as the growing awareness
of the importance of nutrition in patient care has emerged5.
This paper will review the major common alterations
in body composition during critical illness and identify stress-induced
changes in the various body compartments and electrolytes. A further
critical question is whether therapeutic and/or nutritional efforts
influence beneficially the variety of alterations in critically-ill
patients.
Body composition
changes associated with trauma, sepsis and malnutrition
A meaningful discussion about disease-induced alterations
of body composition needs a understanding of normal compartmentalization.
In healthy volunteers the body is composed of two distinct non-osseous
compartments: fat mass and fat-free body mass, the latter being composed
of water, protein, minerals and glycogen 5,7. Normal values
of these components for a typical healthy adult are given in Figure
1. In normal individuals, adipose tissue composes 25-35% of body weight
extracellular fluid 30-40%, and body cell mass (BCM) (the actively
functioning protein-rich tissue and its intracellular fluid) 25-40%.
It is helpful to further subdivide both body water (plasma-intravessel,
interstitial and intracellular water) and body protein (muscle protein,
visceral protein and structural protein).
A series of well-described changes that alter body
composition is associated with stress and malnutrition. The most notable
initial change is an increase in the extracellular fluid component,
accompanied by sodium retention and probably weight gain7.
On the other hand BCM may gradually shrink with stress, resulting
in loss of weight and body fat. As a general rule these patients have
a simultaneously increased hydration of their fat-free body mass due
to the increase in extracellular water8.
Figure 1. Normal body composition (%) and the
components of weight changes in patients suffering from critical illness.
Figures denote percent of body weight. Adopted from Shizgal, 19837
with permission (see journal article).

Trauma-, injury-, sepsis or malnutrition-induced weight
loss is due to the accelerated breakdown of body protein and fat.
Using in vivo neutron activation analysis, tissue composition of the
weight loss was measured two weeks after major abdominal operation
(abdominoperineal excision). The body weight loss was 4.1 kg, composed
of 1 kg protein, 1.3 kg fat and 1.8 kg water9. Protein
and fat-containing tissues can be lost at rates as rapid as 500 g/day,
while the rate of synthesis of lean tissue is approximately only 150
g/day, of which the share of protein corresponds to about 31 g/day.
Body composition changes in patients suffering from critical illness
as compared with normal values are illustrated in Figure 1. With catabolic
illness, there is expansion of the extracellular fluid compartment
and erosion of adipose tissue and BCM7.
In an ongoing study body composition has been measured
by using bio-electrical impedance analysis (BIA) in surviving and
non-surviving septic and multiple trauma patients (Table 1). BIA measurements
were performed daily at a single frequency (50 kHz, 800 m A; Danninger Medical Inc, Columbus/Ohio USA). Resistance and reactance
were measured in triplicate and the mean was used for computerized
calculation BCM, lean body mass (LBM) and extra cellular mass (ECM).
The formulae of McDougall and Shizgall9a were used for
these calculations. In these formulae, ECM is derived from the difference
between LBM and BCM and ECM includes bone minerals. Compared with
healthy subjects, ECM was increased at admission, whereas BCM was
maintained in trauma and decreased in septic patients. The initial
increase of ECM was more accentuated in non surviving patients and
the considerable elevation of ECM was not fully accounted for by a
corresponding elevation of LBM. At discharge a tendency for normalization
of ECM was observed in surviving patients, while with sepsis BCM remained
low. In non-surviving patients a further marked elevation of the ECM
(sepsis 17.8 kg and multiple trauma 19.4 kg) and a decline of BCM
(2.0 kg and 4.5 kg, respectively) were detected. The results thus
indicate that a considerable portion of BCM had been lost. Since the
observed water shifts apparently did not directly affect the size
of BCM, it may be assumed that the diminished mass seen was due to
loss of fat and preferentially to substantial amounts of intracellular
protein. The poor correlation between body water changes and the size
of LBM during stress may explain why it is difficult to relate metabolic
and biochemical alterations per unit of LBM.
Table 1. Body composition in surviving and
non-surviving septic and multiple trauma patients (n) receiving TPN
over 8-20 days Energy was given according to the actual requirements
as measured by indirect calorimetry in form of equal amounts of glucose
and fat. Amino acids 2.0 g per kg body weight were provided daily.
All patients were mechanically ventilated Non-surviving patients developed
one or more multi-organ failure (MOF). Values are given as Mean ± SEM (Fürst P & Leweling H,
unpublished).
|
Normal
|
Septic
|
Multiple trauma
|
Mass |
(8)
|
Surviving (6)
|
Non-surviving (8)
|
Surviving (20)
|
Non-surviving (7)
|
|
|
initial
|
discharge
|
initial
|
prior to death
|
initial
|
discharge
|
initial
|
prior to death
|
LBM, kg |
57.6± 130
|
59.6± 4.9
|
55.4± 8.9
|
68.7± 14.1
|
78.6± 24.2
|
61.8± 10.2
|
57.0± 10.3
|
67.3± 8.8
|
82.2± 12.3
|
BCM, kg |
26.5± 0.75
|
22.0± 3.1*
|
19.9± 2.0
|
25.6± 6.4
|
23.7± 5.8
|
26.5± 5.5
|
25.1± 5.4
|
28.1± 6.9
|
23.6± 4.7
|
ECM, kg |
31.1± 0.70
|
37.5± 3.6*
|
35.5± 7.8
|
43.1± 13.7
|
54.9± 23.8
|
35.3± 7.0
|
31.9± 7.8
|
39.2± 8.3
|
58.6± 14.4
|
LBM = lean body mass; BCM = body cell mass; ECM =
extracellular mass. *Differs significantly from normal (P<0.05).
During stress, injury and infection, fat is the major
source of energy10; about 50% of the requirement being
covered via catecholamine-induced cAMP mediated lipolysis11.
It is notable that the net loss of body fat is associated with a simultaneous
interstitial accumulation of lipids preferentially in muscle tissue.
Interstitial fat has been measured in muscle biopsy specimens in patients
suffering from various catabolic diseases as shown in Table 2. The
data suggest that interstitial fat may increase multifold per unit
of muscle fat-free dry mass12-14. The increment in injury
or sepsis corresponds to not less than 28 g, and in severe burns 36
g, of interstitial lipid per kg muscle or about 700-1000 g in the
whole body, assuming muscle mass is 40% of the body weight and a uniform
allotment of the lipids. Roth and co-workers observed exceptional
degeneration of muscle tissue in two non-surviving burned patients
(Fig 2). The lipid portion in muscle was 53% and 37% of the wet-weight,
associated with a markedly decreased share of muscle water14.
Table 2. Muscle water and electrolytes in critically
ill-patients13 and in patients with liver cirrosis14.
All values are calculated with 100 g fat-free solids (FFS) as the
basis of reference (Mean ± SEM). Significance with normal
values *P<0.05; **P<0.01; ***P<0.001.
|
Normal
|
Multiple injury and bums
(25)
|
Sepsis on admission
|
Subsequent sepsis
|
Convalescence
|
Liver cirrosis
|
|
(85)
|
8 day
|
30 day
|
(17)
|
day 10 (6)
|
day 20 (6)
|
(7)
|
Total water, ml |
336± 1.50
|
364± 5.8***
|
397± 7.2***
|
359± 5.0***
|
366± 13***
|
351± 0.3*
|
413± 22.0***
|
extra cellular |
47± 1.40
|
100± 8.0***
|
127± 11.1***
|
79± 9.0***
|
88± 20*
|
89± 17*
|
158± 24.5***
|
intracellular |
289± 1.50
|
267± 4.6***
|
275± 6.3*
|
281± 7.0
|
278± 10*
|
261± 15*
|
256± 4.2***
|
Fat, g |
4.4± 0.22
|
23.1± 1.9***
|
13.8± 0.91***
|
21.6± 3.3***
|
20.2± 4.2**
|
12.0± 2.1***
|
22.9± 5.1
|
Sodium, mmol |
9.8± 0.21
|
16.5± 5.0***
|
19.5± 1.4***
|
14.0± 1.5**
|
15.5± 2.0*
|
18.2± 2.1**
|
|
Chloride, mmol |
6.6± 0.15
|
12.9± 0.7***
|
16.9± 1.4***
|
11.0± 0.9***
|
12.1± 2.2
|
12.3± 1.7**
|
|
Potassium, mmol |
45.9± 0.20
|
40.0± 0.5***
|
40.0± 1.1***
|
44.5± 0.3***
|
43.9± 0.6**
|
44.4± 0.6***
|
|
Magnesium, mmol |
4.3± 0.04
|
3.9± 0.0***
|
3.7± 0.1***
|
4.3± 0.2
|
4.0± 0.3
|
4.0± 0.2*
|
|
Figure 2. Water and fat content in muscle tissue
in two surviving and two non-surviving patients as compared with the
normal distributions. In the exceptionally degenerated muscle tissue
from the non-surviving patients a considerable accumulation of the
interstitial (extracellular) fat and marked decreased share of the
muscle water was found. From Roth et al., 199114 with permission.
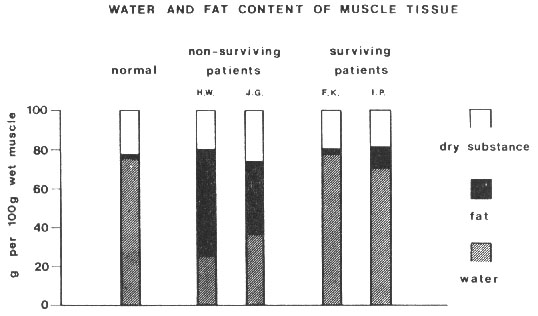
Thus it appears that, in critically-ill patients,
increased lipolysis and augmented free-fatty acid flux coexist with
decreased peripheral utilization of available fatty acids. This biochemical
event is presumably due to diminished intracellular oxidative capacity
caused by energy deficit of the sick cell13,15-17. In the
light of these results it is remarkable that increased fat and decreased
water contents are not shown by BIA in terminal patients. One may
speculate that in these patients their substantial interstitial fat
was not detected and thus that the redistribution of lipids to the
extracellular compartment was simply accounted for by ECM. The redistribution
of body fat compartments in critical and terminal illness, though
of essential metabolic and quantitative importance, has not yet been
acknowledged in the evaluations of body composition changes during
injury and infection.
About two-thirds of total body water and 96% of body
potassium are intracellular. The intracellular content of magnesium
is also high in comparison with the content in the extracellular fluid.
Therefore, changes in muscle water distribution and electrolyte composition
in regions not adjacent to areas of damage or surgical injury should
give valuable quantitative information about the generalized tissue
and cell response to stress and infection. The most consistent effects
of surgical trauma are increases in muscle water, sodium and chloride,
whereas the predominant intracellular electrolytes potassium and magnesium,
are less affectedl2,l8-20. The alterations seen in muscle
composition following severe injury and sepsis are similar to those
observed in postoperative trauma, but apparently more pronounced.
Additionally, cell protein, and the major intracellular kations, potassium
and magnesium contents are decreasedl3,18 (Table 2). An
evaluation of the decreased protein and increased extracellular water
contents, and the changes in electrolyte concentrations, suggest a
correlation of these variables with loss of cell content rather than
of cell numberl3,20. This assumption is supported by conclusions
drawn from BIA suggesting loss of intracellular protein in critically-ill
patients (Table 1).
The findings seen in muscle tissue are consistent
with the well-known effect of trauma on retention of water and sodium,
determined by metabolic balance studies21-23 and body composition
studies using tracer techniques24,25. The finding of an
increase in extracellular muscle water is of interest, because it
proves that fluid retention occurred in non injured portions of the
body. Since muscle is the largest component of lean tissue, modest
changes in muscle can have quantitative significance in explaining
changes in the whole body. The results shown in Table 2 suggest that
extracellular water in muscle tissue increases to two to three times
the normal value with uncomplicated course of severe illness, which
may represent no less than 150-200 ml excess water/kg muscle, or about
5 l of fluid, if uniformly distributed in the skeletal musculature.
As shown in Table 1, patients may exhibit considerable higher excess
of ECM when sepsis and multiple injury is complicated by multiple
organ failure.
It is generally assumed that convalescence from acute
illness or injury includes diuresis of any fluid retained during the
initial days of illness or injury. Therefore, it is surprising that
abnormal water and Na+ retention in muscle persists for
as long as 30 days after injury or sepsis (Table 2), and that high
ECM is measured at discharge following sepsis and multiple trauma
(Table 1), at a time when most patients would be expected to be beyond
a period of diuresis26. In further support of a prolonged
abnormality of muscle electrolyte metabolism are the observations
that the contents of muscle K+ were low in late convalescence
and that, in many patients, muscle magnesium was low on the day 30,
in relation to fat-free solids or to muscle potassium (Table 2). It
is indeed difficult to assess whether the decreased content of potassium
and magnesium is a sign of true intracellular depletion of these ions
or simply an effect of a decreased cellular mass in relation to total
solids. On the other hand, both events may be expected to occur in
depletion or in hypercatabolic situations as a result of ion leakage
across the cell membrane and due to catabolic breakdown of cell protein.
Nevertheless, these observations indicate that severe trauma and sepsis
exert prolonged effects on tissue electrolyte and water metabolism,
a phenomenon which is usually not considered, although it may seriously
influence metabolism, substrate utilization and indeed body composition
in pathological conditions.
Several factors must be considered as possibly related
to the aetiology of the observed composition changes. Prolonged periods
of rest and thus inactivity certainly contribute to muscle protein
loss and consequently may influence the water and electrolyte contents.
The classic work by Deitrick and co-workers suggests inactivity to
be associated with muscle wasting, even if nutrition is adequate27,
while semi-starved but active muscle is preserved. Clearly, bedrest
for four days did not reveal most of the alterations in muscle, which
were observed three to four days after operation or injuryl8,28.
Except for the slight net loss of potassium, there were no significant
changes in water and electrolytes. This suggest that the changes occurring
following injury cannot be explained in the basis of inactivity associated
with semi-starvation and are probably related to trauma.
It is possible that cellular energy metabolism may
be related to the findings since decreased contents of energy-rich
phosphates and reduced energy charge potential are common findings
after injury and in critically-ill patientsl5-l7,29,30.
Accordingly, ATP and adenine nucleotides are decreased after critical
illness and remained so even at day 3031. Hence, there
is a possibility that the skeletal muscle cells remain metabolically
deranged to the extent that a normal intracellular K+ content
cannot be maintained. A low muscle magnesium level is consistent with
this hypothesis. Up to 80-85% magnesium is known to be bound to adenine
nucleotides in the cell32. Thus one would expect a low
tissue magnesium content when ATP, ADP and total adenine nucleotides
are low. Consequently, a significant correlation between ATP/ADP ratio
and intracellular magnesium has been demonstrated in the elderly,
and in patients with respiratory and liver failure 16,33-36.
There is, finally, the possibility that nutrition
was inadequate with regard to one or more specific, nutrients, contributing
to the observed results.
The effects
of nutritional therapy on body composition
For purposes of nutritional treatment, one may divide
critically-ill patients into four somewhat arbitrary groups: (1) malnourished,
remote from injury or sepsis; (2) previously well-nourished, acutely
injured or septic; (3) malnourished, injured or septic who respond
to nutritional efforts, and (4) malnourished, injured or septic patients
who cannot respond appropriately to nutrition37,38.
The long-term goal of nutritional therapy in malnourished,
critically-ill patients without injury or sepsis is to restore BCM.
The composition of weight loss due to chronic starvation, eg in anorexia
nervosa, is equally divided between BCM and fat. With fasting or acute
starvation the composition of weight loss is about 70% of BCM and
30% fat38,39. Undoubtedly, aggressive enteral or parenteral
nutrition, with high intakes of energy and protein, may lead to gain
in both protein and fat in depleted critically-ill patients during
short-term therapy40.
Nutritional treatment of acutely-ill, injured, septic
or burned patients, with or without malnutrition, is associated with
severe problems. The obvious goal for these patients is to minimize
losses of BCM in order to counteract the accelerated net breakdown
of body protein. However, serial measurements of body composition41
and substrate flux studies42 indicate that it is extremely
difficult to maintain or replenish body protein during stress. Weight
gain may be observed in patients treated with iv nutrition but is
more likely to be due to water retention and glycogen deposition than
true gain in cellular protein43, 1 g of glycogen obligating
about 3 g of water44,45. In critically-ill septic patients
10 days of parenteral nutrition with 2700 kcal and 130 g amino acids
per day resulted in a considerable loss of body weight (6.2 kg). The
share of protein loss was estimated to yield 1.5 kg, corresponding
to 12.5% of the BCM, while body fat increased by 2.2 kg. Changes in
body composition that occurred in patients with gastrointestinal dysfunction
receiving TPN over a two-week period are illustrated in Figure 3.
It is obvious that most of the weight gain can be accounted for in
terms of water and, to a lesser extent, to fat. All patients but two
revealed loss of protein, on average 1.1 kg5.
Figure 3. Changes in body composition that
occurred in 20 patients with gastrointestinal dysfunction receiving
TPN over a 2-week period. The broken lines indicate the maximum difference
between the measurements, which can with 95% probability to be attributed
to measurement error alone. Most of the weight gain can be accounted
for in terms of water. All patients, but two, revealed loss of protein.
From Hill & Beddoe5 with permission (see journal article).
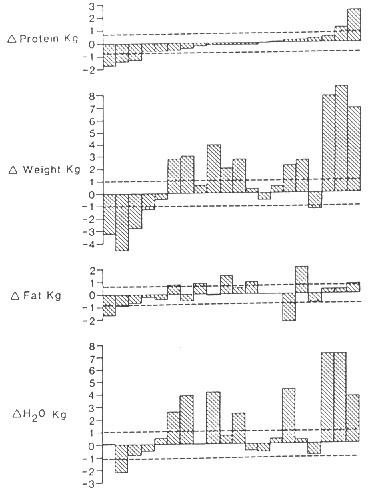
In a current investigation the effect of TPN on body
composition was evaluated in 14 medical and surgical intensive care
patients receiving energy corresponding to 1.6 RME and 1.5 g amino
acids per kg ideal body weight (Table 3). BIA was performed at onset
of treatment and after 2 weeks on TPN. In comparison with results
obtained in healthy controls a loss of body weight (20%) and BCM (17%)
was observed. With the aggressive nutritional therapy employed, BCM
remained essentially unchanged at completion (Fürst & Leweling,
unpublished). In other patients with a wide variety of gastrointestinal
diseases body weight was similarly reduced by 20% and total body protein
by 21%5 (Beddoe et al., unpublished), compared with the
largest body of data on normal humans46. In critically-ill
patients a strong relationship between changes in BCM and energy and
protein intakes were demonstrated47. Nutritional therapy
with enteral or parenteral nutrition was directed toward maintenance
of BCM and a restoration of stress or malnutrition-induced depletion
of BCM in agreement with earlier claims7. Accordingly,
an increase in BCM in response to nutritional efforts is only possible
in the presence of a pre-existing malnutrition. This postulate conforms
with the finding that the repletion value of BCM was correlated with
the degree of malnutrition, the Nae/Ke ratio
and with the amounts of nutrient infused48.
As described, the alterations seen in muscle composition
following post-operative injury12,20 appear to be similar
to those found in critically-ill patients13,18,19 and in
patients with multiple trauma13,31; the changes were related
to the severity of injury. It appears that the different nutritional
regimens used were without influence on the concentration changes
observed in response to trauma. Neither different amino acid composition
nor hypocaloric nor normocaloric supply of energy revealed differences
in post-traumatic muscle composition12. In critically-ill,
septic or burned patients similar findings were obtained regardless
of whether the energy intake was high or low, the glucose intake was
high or low, and whether lipid was included in the intake31.
The changes in muscle water and electrolytes were essentially similar
whether amino acids were given or not during the first eight I days
after the trauma13.
Table 3. The effect of TPN in 14 medical and
surgical intensive care patients (5 F and 9 M, age range 21-58, mean
body weight 79.3 ± 2.2) at onset and at completion of aggressive nutritional treatment
(Energy: 1.6 RME and amino acids 1.5 g/kg ideal body weight). The
results are compared with those obtained in 18 apparently healthy
controls (age range 22-67, mean body weight 79.3 ± 2.2) (Fürst P & Leweling H, unpublished).
|
Normal
|
Critically ill patients
|
|
|
onset of TPN
|
completion of TPN
|
LBM kg |
57.6 ± 1.3
|
48.7 ± 7.2
|
48.8 ± 7.4
|
BCM, kg |
26.5 ± 0.75
|
21.9 ± 3.6
|
22.0 ± 4.0
|
ECM, kg |
31.1± 0.7
|
26.8± 4.7
|
26.7± 4.7
|
LBM lean body mass; BCM body cell mass; ECM extracellular
mass.
Although malnutrition is a severe complication in
critically-ill patients, it is not the primary cause of their illness
and nutrition is a necessary adjunct to the primary therapy. The majority
of these patients with adequate therapy and nutrition will finally
respond with improved N-balance and recovery despite compromised body
composition38. However, there is a subgroup of patients
with chronic sepsis or multiple-organ failure who are unable to respond
to nutrition, remain in negative N balance, undergo pathologic alterations
in body composition and subsequently die. In malnourished injured
and/or septic patients complicated with multi-organ failure, the considerable
loss of body weight and body protein are consistent findings, despite
maximum iv nutritional intakes and positive energy balance41.
Increased release of catabolic hormones and reduction of intracellular
glutamine pool appear to be the hallmark of the response to injury
and infection59-52. However, the underlying mechanisms
for the alterations seen in body composition, and the association
of these changes with the metabolic responses to catabolism and subsequent
wasting, remain unclear53.
A fascinating current hypothesis has been proposed
emphasizing the essential importance of the cellular hydration state
as a determinant of protein catabolism in health and disease54.
It is postulated that an increase in cellular hydration (swelling)
acts as an anabolic proliferative signal, whereas cell shrinkage is
catabolic and antiproliferative55,56. Undoubtedly, hormone-induced
changes in cellular hydration are seen as another 'second messenger'
of hormone action57,58. Moreover, concentrative amino acid
transport systems in the plasma membrane may also act as a transduction
signal set-up, modifying cellular function by changing the hydration
state. Low activities of amino acid transporter, Na+/H+
antiport or Na-K-2Cell co-transporter, and opening of K+
channels under the influence of altered nutrition, cytokines and free
radicals, can all contribute to cellular shrinkage, which acts as
the common end-path, triggering net protein breakdown58-60.
The hypothesis contemplates the activity of the ion and substrate
transport system and, to a I lesser extent, the size of the extracellular
space. Indeed, expansion of extracellular water is carefully considered
by the physician, whereas changes of intracellular water is largely
ignored. Liver or muscle cells swell as much as 10-12% within two
minutes under the influence of glutamine and increased cellular hydration
is maintained as long as the amino acid is present59, supporting
the notion that glutamine stimulates protein synthesis61-63.
Thus, changes in cellular hydration state may be the variable linking
muscle glutamine content with protein turnover and, because of the
large muscle mass, whole body nitrogen balance. Data from previous
studies of the relation between intracellular glutamine content and
catabolism in patients with various underlying disorders enabled the
evaluation of the relation between muscle cell water content and whole
body nitrogen balance, showing an inverse relation (Fig. 4). The concentrative
uptake of gluatime into muscle and liver cells would be expected to
increase cellular hydration, thereby triggering a protein anabolic
signal. Indeed, preparations containing glutamine dipeptides 64,65
may facilitate aggressive therapeutical inter-ventions by improving
the cellular hydration state and subsequently modifying or reversing
catabolic changes66,67.
Although it may be possible to improve the course
of critical illness with some more optimal form of nutrition than
is presently known, it seems however unlikely that, without improved
treatment of the primary disease, improved nutrition will be decisive
in influencing body composition and recovery.
Figure 4. Whole body nitrogen balance and cellular
hydration of skeletal muscle. A = healthy subjects (n = 17); other
subjects are patients suffering from liver tumours = B (n = 5); polytrauma
day 2 = C and day 9 = D after trauma (n - 11 ); acute necrotizing
pancreatitis = E (n = 6); bums = F (n = 4). Skeletal muscle water
was assessed in biopsy specimens from m. quadriceps femoris and the
extra/intracellular distribution was calculated by the chloride method,
assuming normal membrane potential of -87.2 mV. For references cf
Bergström et al., 1981 and 198712,13. From Häussinger et
al.54 with permission.
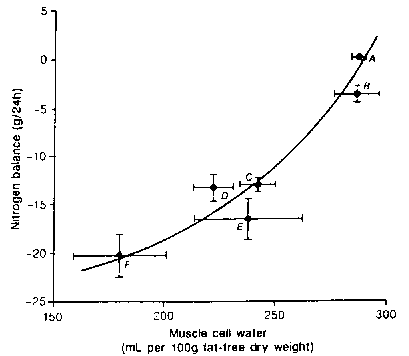
References
1. Beisel WR. Metabolic response to infection. Ann
Rev Med 1975; 26:9-20.
2. Wilmore DW. Catabolic illness. Strategies for enhancing
recovery. N Engl J Med I 991; 325:695-702.
3. Cerra FB. Hypermetabolism, organ failure, and metabolic
support. Surgery 1987; 101:1-14.
4. Cheney CL, Lenseen P, Aker SN, et al. Sex differences
in nitrogen balance following marrow grafting for leukemia. J Am Coll
Nutr 1987; 6:223-230.
5. Hill GL, Beddoe AH. Dimensions of the human body
and its compartments. In: Kinney JM, Jeejeebhoy KN, Hill GL, Owen
OE, eds. Nutrition and metabolism in patient care. Philadelphia, London,
Toronto, Montreal, Sydney, Tokyo: Saunders, 1988:89-118.
6. Elia M, Jebb SA. Assessment of body composition:
Research techniques and bedside methods. SA J Clin Nutr 1990;3:21-26.
7. Shizgal HM. Body composition. In: Fischer SE, ed.
Surgical nutrition. Boston, Toronto: Little, Brown and Company, 1983:
6-17.
8. Moore FD, Boyden CM. Body cell mass and limits
of hydration of the fat free body: their relation to estimated skeletal
weight. Ann NY Acad Sci 1963; 110:62-71.
9. Hill GL, McCarthy ID, Collins JP, et al. A new
method for the rapid measurement of body composition in critically
ill surgical patients. Br J Surg 1978; 65:732-735.
9a. McDougall D, Shizgall HM. Body composition measurements
from whole body resistance and reactance. Surg Forum 1986; 37:42-44.
10. Levenson SM, Crowley BS, Seifter E. Starvation.
In: Committee on Pre- and Postoperative Care, American College of
Surgeons, eds. Manual of surgical nutrition. Philadelphia: Saunders,
1975:236.
11. Steinberg D, et al. Hormonal regulation of lipase,
phosphorylase and glycogen synthetase in adipose tissue. Adv Cyclic
Nucleotide Res 1975; 5:549.
12. Bergström J, Fürst P, Holmström B, et al. Influence
of injury and nutrition on muscle water and electrolytes. Effect of
elective operation. Ann Surg 1981; 193:810-816.
13. Bergström J, Larsson J, Nordström H, et al. Influence
of injury and nutrition on muscle water and electrolytes; effect of
severe injury, bums and sepsis. Acta Chir Scand 1987; 153:261-266.
14. Roth E. Muskulärer Elektrolyt- und Wasserstoffwechsel
bei Sepsis. In: Deetjen P, Reissigl H, eds. Intrazellulare Elektrolyte
und ihre therapeutische Beeinflussung. Bibliomed Medizinische Verlagsgesellschaft,
1991 :83-90.
15. Liaw KY, Askanazi J, Michelsen CB, Fürst P, Elwyn
D, Kinney J. Effect of postoperative nutrition on muscle high energy
phosphates. AnnSurg 1982; 195:12-18.
16. Kinney J, Fürst P, Elwyn D. Carpentier YA. The
intensive care patient. In: Kinney I, Jeejeebhoy KN, Hill GL, Owen
OE, eds. Nutrition and metabolism in patient care. Philadelphia al:
Saunders, 1988:656-671.
17. Fürst P. Bioenergetics of human skeletal muscle
in normal and pathological conditions. Rivista Italiana Parenterale
ed Enterale 1993; 11: 1-11.
18. Bergström J, Fürst P, Chao L, et al. Changes of
muscle water and electrolytes with severity of trauma. Acta Chir Scand
1979; 494 (Suppl):139-141
19. Vinnars E, Fürst P, Gump FE, Kinney JM. Influence
of trauma and sepsis on water and electrolytes of human muscle tissue.
Surg Forum 1975; 26:16-18.
20. King RF, Collins JP, Morgan DB, Hill GL. Muscle
chemistry of critically ill surgical patients and the effects of a
course of intravenous nutrition. Br J Surg 1978; 65:495-498.
21. Doty DB, Hufnagel JV, Mosely RV. The distribution
of body fluids following haemorrhage and resuscitation in combat casualties.
Surg Gynecol Obstet 1970; 130:453-458.
22. Elwyn DH, Bryan-Brown CW, Shoemaker WC. Nutritional
aspects of body water dislocation in postoperative and depleted patients.
Ann Surg 1975; 182:76.
23. Shoemaker WC, Bryan-Brown CW, Quigley L, et al.
Body fluid shifts in depletion and poststress states and their correction
with adequate nutrition. Surg Gynecol Obstet 1973; 136:371.
24. Pluth JR, Cleland J, Meador CK, et al. Effect
of surgery on the volume distribution of extracellular fluid determined
by the sulfate and bromide methods. In: Bergner PE, Lushbaugh EE,
eds. Compartments, pools and spaces in medical physiology. Springfield:
US Atomic Energy Commission, 1967: 217.
25. Bergström J. Muscle electrolytes in man. Scand
J Clin Lab Invest 1962; 14:1-110.
26. Shires GT, Carrica CJ, Baxter CR. Principles in
treatment of severely injured patients. In: Welch CE, ed. Advances
in Surgery. New York: Yearbook Medical,1970:255-324.
27. Deitrick JE, Whedon GD, Shorr E. Effects of immobilization
upon various metabolic and physiologic functions of normal men. Am
J Med 1948; 4:3-8.
28. Askanazi J, Elwyn DH, Kinney JM, et al. Muscle
and plasma amino acids after injury. The role of inactivity. Ann Surg
1978; 188:797-803.
29. Bergström J, Fürst P, Hultman E, Vinnars E. Preliminary
studies of energy-rich phosphagens in muscle from severely ill patients.
Crit Care Med 1976; 4: 197-204.
30. Liaw KY, Askanazi J, Michelsen CB, Kantrowitz
LR, Fürst P, Kinney J. Effect of injury and sepsis on high energy
phosphates in muscle and red cells. J Trauma 1980; 20:755-759.
31. Larsson J, Schildt B, Vinnars E, Lilijedahl SO.
The effect of severe trauma on muscle energy metabolism in man. Acta
Chir Scand 1984; 150:611-618.
32. Nanninga LB. Calculation of free magnesium, calcium
and potassium in muscle. Biochim Biophys Acta 1961; 54: 338-344.
33. Gertz I, Hedenstierna G, Hellers G, et al. Muscle
metabolism in patients with chronic obstructive lung disease and acute
respiratory failure. Clin Sci Mol Med 1977; 52:395-403.
34. Moller P, Bergström J, Fürst P, et al. Effect
of aging on energy rich phosphagens in human skeletal muscles. Clin
Sci 1980; 58:553-555.
35. Moller P, Bergström J, Fürst P, Hellström K, Uggla
E. Energy rich phosphagens, electrolytes, and free amino acids in
leg skeletal muscle of patients with chronic obstructive lung disease.
Acta Med Scand 1982;211: 187- 193.
36. Muller, P, Bergström J. Fürst P, Hellström K.
Muscle biopsy studies in patients with moderate liver cirrhosis with
special reference to energy-rich phosphagens and electrolytes. Scand
J Gastroenterol 1984; 19:267-272.
37. Elwyn DH. Nutritional requirements of adult surgical
patients. Crit Care Med 1980; 8:9-20.
38. Elwyn DH. Protein metabolism and requirements
in the critically ill patient. Crit Care Clin 1987; 3:57-69.
39. Insel J, Elwyn DH. Body composition. In: Askanazi
J, Starker P, Weissmann C, eds. Fluid electrolyte management in critical
care patients. Boston: Butterworths, 1986:3-21.
40. Hill GL, Church J. Energy and protein requirements
of general surgical patients requiring intravenous nutrition. Br J
Surg 1984; 71: 1-9.
41. Streat SJ, Beddoe AH, Hill GL. Aggressive nutritional
support does not prevent protein loss despite fat gain in septic intensive
care patients. J Trauma 1987; 27:262-266.
42. Loder PB, Smith RC, Kee Al, et al. What rate of
infusion of intravenous nutrition solution is required to stimulate
uptake of amino acids by peripheral tissues in depleted patients?
Ann Surg 1990;211:360 368.
43. Hill GL, Bradley JA, Smith RC, et al. Changes
in body weight and body protein with intravenous nutrition. JPEN 1979;
3: 215-218
44. Bergström J, Beroniade V, Hultman E, Roch-Nordlund
AE. Relation between glycogen and electrolyte metabolism in human
muscle. In: Kruck SH, ed. Symposium uber Transport and Funktion intracellularer
Elektrolyte. Munchen: Urban & Schwarzenberg, 1967: 108-115.
45. Chan STF, Johnson AW, Moore MH, et al. Early weight
gain and glycogen-obligated work during nutritional rehabilitation.
Hum Nutr Clin Nutr 1982; 36:223-232.
46. Cohn SH, Vartsky D, Yasumura S, et al. Compartmental
body composition based on total body nitrogen, potassium and cal cium.
Am J Physiol 1980; 39:E524-E530.
47. Robert S, Zarowitz BJ, Hyzy R, Eichenhorn M, Peterson
EL, Popovich Jn Jr. Bioelectrical impedance assessment of nutritional
status in critically ill patients. Am J Clin Nutr 1993; 57:840-844.
48. Shizgal HM. Protein requirements with total parenteral
nutrition. Surg Forum 1978; 24:60-62.
49. Smith R, Williamson DH. Biochemical effects of
primary injury. Trends Biochem Sci 1983; 4: 142-143.
50. Rennie MJ. Muscle protein turnover and wasting
due to injury and disease. Br Med J 1985; 41 :257-264.
51. Fürst P. Intracellular muscle free amino acids
-- their measurement and function. Proc Nutr Soc 1983; 42:451-462.
52. Fürst P. Regulation of intracellular metabolism
of amino acids. Arvid Wretlind Lecture. In: Bozetti F, Dionigi R,
eds. Nutrition in cancer and trauma sepsis. Basel: Karger,1985:21-35.
53. Mortimore GB, Poso AR. Intracellular protein catabolism
and its control during nutrient deprivation and supply. Ann Rev Nutr
1987; 7:539-564.
54. Häussinger D, Roth E, Lang F, Gerok W. Cellular
hydration state: an important determinant of protein catabolism in
health and disease. Lancet 1993; 341:1330 1332.
55. Häussinger D, Hallbrucker C, von Dahl S, et al.
Cell volume is a major determinant of proteolysis control in liver.
FEBS Lett 1991; 283:470-472.
56. Stoll B, Gerok W, Lang F, Häussinger D. Liver
cell volume and protein synthesis. Biochem J 1992; 287:217-222.
57. von Dahl S, Hallbrucker C, Lang F, Häussinger
D. Regulation of liver cell volume by hormones. Biochem J 1991; 280:
105-109.
58. Häussinger D, Lang E Cell volume and hormone action.
Trends Pharmacol Sci 1992; 13:371-373.
59. Häussinger D, Lang F, Bauers K, Gerok W. Interactions
between glutamine metabolism and cell-volume regulation in perfused
rat liver. Eur J Biochem 1990; 188:689-695.
60. Häussinger D, Lang E Cell volume in the regulation
of hepatic function: a new mechanism for metabolic control. Biochim
Biophys Acta 1991; 1071:331-350.
61. MacLennan P, Smith K, Weryk B, Watt PW, Rennie
MJ. Inhibition of protein breakdown by glutamine in perfused rat skeletal
muscle. FEBS Lett 1988; 237: 133- 136.
62. Jepson MM, Bates PC, Broadbent P, Pell JM, Millward
DJ. Relationship between glutamine concentration and protein synthesis
in rat skeletal muscle. Am J Physiol 1988; 255: E166-E172.
63. Barua JM, Wilson E, Downie S, Weryk B, Cuschieri
A, Rennie MJ. The effect of alanyl-glutamine peptide supplementation
on muscle protein synthesis in post-surgical patients receiving glutamine-free
amino acids intravenously. Proc Nutr Soc 1992; 51:104A.
64. Fürst P. New parenteral substrates in Clinical
Nutrition Part 1. Introduction. New substrates in protein nutrition.
Eur J Clin Nutr 1994;48:607-676.
65. Fürst P, Albers S, Stehle P. Glutamine-containing
dipeptides in parenteral nutrition. JPEN 1990; 14:118S-124S.
66. Stehle P, Zander J, Mertes N, et al. Effect of
parenteral glutamine peptide supplements on muscle glutamine loss
and nitrogen balance after major surgery. Lancet 1989; i:231-233.
67. Hammarqvist F, Wernerman 1, Von der Decken A,
Vinnars E. Alanyl-glutamine counteracts the depletion of free glutamine
and the postoperative decline in protein synthesis in skeletal muscle.
Ann Surg 1990; 212:637 644.

Copyright © 1995 [Asia Pacific Journal of Clinical
Nutrition]. All rights reserved.
Revised:
January 19, 1999
.