Asia Pacific J Clin Nutr (1993) 2, Suppl 1, 5-13
Cellular functions of ascorbic
acid: a means to determine vitamin C requirements
Mark
Levine MD, Kuldeep R Dhariwal
PhD, Philip W. Washko PhD, DMP, Richard W. Welch PhD, and Yaohui Wang MD
Laboratory of Cell Biology and Genetics,
National Institute of Diabetes and Digestive and Kidney Diseases,
National Institutes of Health, Bethesda, MD 20892, USA.
Optimal ascorbic acid (vitamin C) requirements in
humans are unknown. In situ kinetics is a biochemical approach to
determine requirements for vitamin C and other vitamins. In situ
kinetics requires that cellular functions of ascorbic acid are characterized.
Vitamin-C-dependent cellular reactions are directly related to vitamin
C concentrations inside and outside cells. By coupling intracellular
and extracellular functions of ascorbic acid to vitamin concentration,
in situ kinetics provides a novel approach to determining vitamin
C requirements.
Introduction
Ascorbic acid (vitamin C) is essential for human health.
Without ascorbic acid, the deficiency disease scurvy occurs and is
fatal if untreated. The amount of ascorbic acid necessary for maintaining
health is unknown. This review will focus on how this problem can
be answered by understanding cellular functions of ascorbic acid.
Knowledge of vitamin C and cellular function may play a central role
in determining the dietary allowance.
The current recommended dietary allowance (RDA) for
ascorbic acid is 60 mg in the United States and 30 mg in Australia
(recommended dietary intake, RDI). The RDA is based on three parameters.
The first is prevention of the deficiency disease scurvy, as described
in the Iowa prison studies1-4. Five prisoners were given
ascorbic-acid-free diets for months, with or without small doses of
ascorbic acid. Signs and symptoms of scurvy were prevented when as
little as 10-12 mg of ascorbic acid was ingested. To provide reserves
to prevent scurvy, multiples of this ingestion quantity were selected
as goals for dietary ingestion; for example, six-fold more than the
minimum 10 mg was chosen in the US5. In countries where
the quantity is lower, such as Greece and Australia, the recommendation
can be considered a minimum amount to prevent scurvy in most people.
A second parameter in suggesting a dietary recommendation
was based on urinary excretion of vitamin C. The hypothesis was that
once urinary excretion occurred, body stores of the vitamin were saturated.
From the Iowa prisoners, urinary excretion of vitamin C occurred when
ingestion was approximately 60 mg daily1,3,4. This proposal
was re-examined in outpatients believed to be ingesting no dietary
ascorbic acid6. These volunteers were given an ascorbic
acid dose plus radioactive ascorbic acid tracer to determine catabolism,
compartmentalization, excretion, and body pool size. Urinary excretion
occurred at approximately 60 mg daily.
Based on this latter study6, and others
similar to it3,4, catabolism was chosen as a third parameter
of vitamin C requirements5. Catabolism was estimated
to be approximately 60 mg daily; ingestion of 60 mg would maintain
a body pool size of 1500 mg. A larger body pool size was deemed unnecessary
by the US Food and Nutrition Board5.
Thus, the RDA is based on preventing signs and symptoms
of scurvy, achieving the urinary threshold for vitaming C excretion,
and replacing catabolized vitamin C. At 60 mg daily, scurvy will be
prevented for at least one month if vitamin C ingestion suddenly ceased.
At 60 mg daily, catabolic losses will be replaced, and a body pool
size is maintained which is considered to be adequate. In other countries
such as Australia and Greece, 30 mg is chosen as a dietary intake,
and this too will prevent scurvy but without as large a body pool
size.
Unfortunately, this approach to vitamin C requirements
is unreliable. There are a number of problems with the experiments
themselves. In the Iowa prisoner studies1-4, the assay
for ascorbic acid was neither specific nor sensitive. The assay used
will overestimate ascorbic acid at low concentrations7.
Consistent with this problem, signs and symptoms of scurvy were reported
at plasma concentrations £ 12 m m. This is unusually high compared
to more recent information, where concentrations of 5 m M were reported without signs or symptoms of scurvy8. The
time reported to achieve plateau plasma concentrations at the repletion
dose of 66 mg was substantially prolonged for a water-soluble substance
and is very difficult to explain, suggesting an experimental artifact.
The use of radiolabel as an index of ascorbic acid excretion could
be misleading, as the radiolabel could degrade either during administration
or during assay. In the later studies with tracer5, outpatient
volunteers were studied whose diets were not known with certainty.
If ingestion is not clear, interpretation is very difficult since
it is not certain what amount of vitamin C the volunteers really received.
The identity of the radiolabel excreted in urine was not verified
as ascorbic acid, making it difficult to truly calculate body pool
size and to calculate catabolism. In addition, catabolism estimates
and body pool size can be functions of the ingested dose. Estimates
may be misleading if low but not high doses are investigated, as occurred
in these studies9.
Even more important, the conceptual approach to the
current RDA may be incorrect9. First, the RDA and RDI area
based on a minimum amount to prevent a deficiency disease, scurvy.
But it may well be a flawed concept that a minimum amount is equivalent
to an ideal amount. Second, it is only hypothesis that, when urinary
excretion of ascorbic acid occurs, body pools are of satisfactory
size. Since urinary excretion indicates saturation for some substances
but not others, it may be inappropriate to assume urinary excretion
is equivalent to saturation.
There are other suggestive, although less compelling,
reasons to question current recommendations. Our paleolithic forbears
were estimated to have ingested approximately 400 mg ascorbate10.
Ingestion of ascorbate can be as much as 500 mg on a modern diet rich
in fruit and vegetables, without supplements. Thus, past and present,
the vitamin is readily available in foods. Ingestion of 60 mg but
not higher amounts would be good if the vitamin were toxic. However,
vitamin C is remarkably safe. There are few concerns for toxicity,
as shown in Table 19,11-15. The greatest concern is for
excess iron absorption in patients with iron overload, as in thalassemia,
transfusion overload, or hemochromatosis. Diarrhea can occur at single
doses greater than 3-4 grams; however, there are no sound reasons
to ingest such doses. Although other toxicities have been suggested,
the evidence doesn't substantiate the claims (Table 1). Therefore,
vitamin C does not have a narrow window from therapeutic safety to
toxicity window as is true for many drugs and for fat-soluble vitamins
A and D.
Table 1. Ascorbic acid toxicities and misconceptions
of ascorbic acid toxicities9,11.
Vitamin C Toxicity
- Diarrhea: >3-4 grams at once orally
- Iron absorption: Hemochromatosis, thalassemia
major, sideroblastic anemia.
- False negatives for occult blood in stool
- ??? Oxalate hypersecretion and kidney stones;
Possible for doses greater than 4 grams daily
- ??? Hyperuricosuria: Intravenous doses greater
than 2 grams at once
- ??? Hyperoxalemia in dialysis patients
|
Misconceptions of Vitamin C Toxicity
- Rebound scurvy (conditioning)
- Hemolysis
- Mutagenesis
- Venous stasis
- Destruction of vitamin B12 in
food
|
Except for primates, most mammals synthesize ascorbic
aid. Adjusted to the weight of a 70 kg human, these synthetic rates
have been claimed to correspond to 500 6000 mg per day. These data
have been used to suggest that the 60 mg human requirement is very
much underestimated. However, the numbers were obtained by measuring
synthetic rates in liver homogenates containing the enzymes for ascorbate
biosynthesis; these rates were calculated for 24 hours, and then adjusted
for liver weight in different species. Unfortunately, calculations
based on tissue homogenates are not a reliable basis for whole animal
biosynthesis nor for human vitamin requirements9. Nevertheless,
when all of the issues above are considered, there is suggestive evidence
that the RDA is too low.
The dietary recommendations will prevent deficiency,
but do not address an amount for ideal health. How can this amount
be determined? Until recently, techniques to measure optimal vitamin
C ingestion have been indirect. For example, endpoints measured have
been resistance to temperature change, resistance to infection, resistance
to 'stress', or ability to perform exercise9. None of these
endpoints provided information to indicate optimal vitamin C consumption.
Recent epidemiologic evidence has suggested that vitamin C could be
involved in prolonging life or in prevention of atherosclerosis, cancer,
and cataracts16-25. This information is provocative. However,
it is also indirect, or circumstantial. Vitamin C could prevent illness
or prolong life, but something else associated with vitamin C ingestion
and not vitamin C itself might explain the findings. In addition,
for many of them the association is not striking. Future epidemiologic
studies could provide more useful information about optimal ingestion,
but current work is not sufficient.
In situ kinetics
What is missing from all of these approaches to determining
vitamin C requirements is biochemical function of the vitamin. Optimal
vitamin requirements could be determined by knowledge of how much
vitamin is required for a specific biochemical function. For this
approach to be useful for requirements, many functions of vitamin
C must be understood. Knowledge of functions for isolated systems
is not enough, however. Functions must be studied where they occur
in cells or tissues in situ (translated directly: in position), not
simply for isolated reactions and/or proteins9,26. Thus,
as a novel approach to requirements, we can learn how much vitamin
is needed for each different function to occur, in situ; for this
reason, the approach is called in situ kinetics.
In situ kinetics has two major components. The first
is to determine how specific biochemical functions are regulated by
different ascorbic acid concentrations in situ. The second is to learn
how ascorbic acid concentrations are achieved in normal humans which
will permit the biochemical reactions to occur. These principles are
described in detail elsewhere27.
Ascorbic acid is an electron donor, or reducing agent.
As such all of its known biochemical actions involve transferring
its electrons; something else is reduced as ascorbate is oxidized.
Transfer of one electron from ascorbic acid yields the unstable free
radical intermediate semidehydroascorbic acid (half time of 10-5
seconds); transfer of a second electron results in dehydroascorbic
acid28. The ascorbate: dehydroascorbic acid couple is reversible;
for example, dehydroascorbic acid can be reduced back to ascorbic
acid by sulfhydryl reagents. However, hydrolysis of dehydroascorbic
acid to diketogulonic acid is irreversible (see Figure 1).
Figure 1. Ascorbic acid's structure and its
oxidation products. Interconversion between ascorbate, semidehydroascorbic
acid and dehydroascorbic acid is reversible. Once the ring is broken,
diketogulonic acid cannot revert to dehydroascorbic acid. Dehydroascorbic
acid may exist in multiple forms; two are shown here for clarity28.
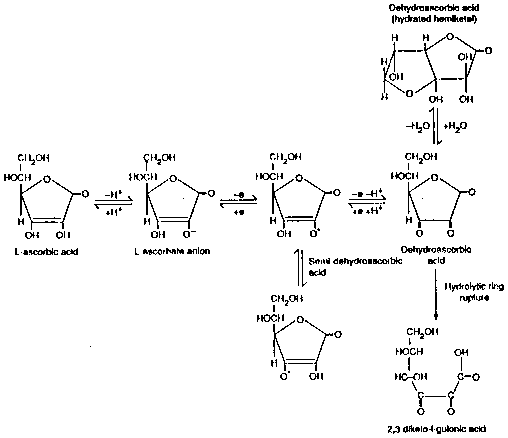
The function of vitamin C as a reducing agent can
be intracellular or extracellular. Intracellular function can further
be divided into enzymatic and non-enzymatic function. Enzymatic intracellular
function occurs when a specific reaction is mediated by an enzyme
and ascorbic acid. Ascorbic acid donates electrons to the enzyme,
in most cases a metal-enzyme complex, which then catalyzes the reaction9.
Non-enzymatic intracellular function is simply the chemical function
of ascorbate as an electron donor, commonly described as anti-oxidant
function.
Likewise, extracellular function can also be divided
into enzymatic and non-enzymatic function. However, while extracellular
enzymatic functions for ascorbate can theoretically exist, none have
been described to our knowledge. Therefore extracellular function
is nonenzymatic or anti-oxidant. A summary of vitamin C's functions
is shown in Table 2 (see9,11 for details).
Table 2. Ascorbic acid's functions9,11,42,64,68-70
(see text for details).
Ascorbic Acid and
Biochemical Function |
|
Intracellular Enzymatic
Function |
|
Proline hydroxylase
(EC 1.14.11.2) |
Collagen synthesis |
Procollagen-proline
2-oxoglutarate 3-dioxygenase (EC 1.14.11.7) |
Collagen synthesis |
Lysine hydroxylase (EC
1.14.11.4) |
Collagen synthesis |
Gamma-butyrobetaine,
2-oxoglutarate 4-dioxygenase (EC 1.14.11.1) |
Carnitine synthesis |
Trimethyllysine-2-oxoglutarate
dioxygenase (EC 1.14.11. 8) |
Carnitine synthesis |
Dopamine beta-monooxygenase
(EC 1.14.17. 3) |
Catecholamine synthesis |
Peptidyl glycine alpha-amidating
monooxygenase (EC 1.14.17.3) |
Peptide amidation |
4-Hydroxyphenylpyruvate
diooxygenase (EC 1.13.11.27) |
Tyrosine metabolism |
Intracellular Non-enzymatic
Function
?Quenching of reactive oxidants
(?neutrophils, monocytes) |
|
Extracellular- Non-ezymatic
Function
?Prevention of LDL oxidation
?Quenching of reactive oxidants
Iron absorption in gastrointestinal tract |
|
Function Uncertain
tissue contains high concentrations |
|
Host defense
B lymphocytes, T lymphocytes, platelets |
|
Endocrine
Adrenal cortex, testes, ovaries, pancreas |
|
Vision
Lens Retina |
|
How these vitamin functions are used for in situ kinetics
is displayed in Figure 2, in which different functions of the vitamin
are expressed in relation to vitamin concentration. Each curve in
the figure can represent a different function from Table 2. The curves
can be thought of as dose response curves, or biochemically as substrate
velocity plots. Toxicity is indicated by a decrease in some functions
at higher vitamin concentrations. The ideal vitamin concentration
may be that which yields maximal function but without toxicity, as
shown in the figure. Optimal vitamin ingestion would be that amount
which provides this vitamin concentration26,27.
Figure 2. Ascorbic acid's functions in relation
to its concentration. Each curve represents a distinct function dependent
on ascorbate. Toxicity for some functions is indicated by decreasing
activity at higher ascorbate concentrations. The curves are theoretical
curves.
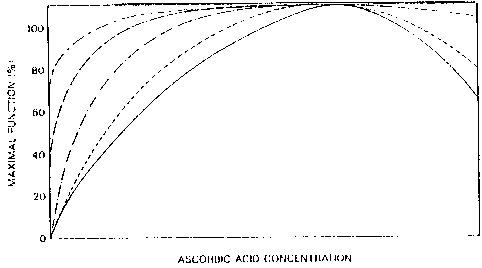
For in situ kinetics, there is a major advantage to
studying intracellular function of ascorbate, especially enzymatic
function. The advantage is that enzyme function can be directly and
specifically related to ascorbate concentration. Ascorbate can be
a co-substrate or co-factor for eight different enzymatic reactions,
all of which occur intracellularly. For these enzymes to have maximal
activity, ascorbate must be the specific electron donor, not other
reducing agents9. Specificity is essential for studying
reaction kinetics. Specificity allows meaningful conclusions about
how much vitamin is necessary for a particular function in situ.
Intracellular non-enzymatic (antioxidant) function
may or may not be specific for ascorbate. If specificity for ascorbate
exists, the same advantages as for enzyme reactions apply. Even if
there is no specificity for ascorbate, the reaction can still have
meaning if it is physiologic. Physiologic relevance can be determined
when the reaction is studied in place within cells (in situ).
However, if isolated rather than in situ reactions
are studied, results can be obtained which might not occur in situ.
This is because vitamin C donates electrons easily; many in vitro
conditions which favor electron transfer may simply have no in vivo
counterpart of physiologic relevance29,30. Vitamin C may
act as an electron donor for isolated non-enzymatic reactions, but
the same reactions may not occur in situ or vitamin C may not be the
electron donor in situ. Similar problems are encountered when the
effects of other reducing substances are tested on isolated reactions
or proteins.
Extracellular function of vitamin C is non-enzymatic,
where vitamin C acts as an antioxidant. It has been very difficult
to study these reactions in situ, and isolated reactions are studied
instead. But as just noted, antioxidant function is difficult to interpret
if only isolated reactions are studied. Vitamin C might act as an
electron donor for isolated reactions but have no relevance for such
reactions in situ. For these reasons, we will concentrate here on
intracellular function, where specificity and physiologic relevance
can be addressed.
Catecholamine
biosynthesis
The catecholamines dopamine, norepinephrine, and epinephrine
are synthesized from the amino acid tyrosine. The enzyme dopamine
beta monooxygenase is a mixed function oxidase that catalyzes the
formation of norepinephrine from dopamine. The isolated enzyme requires
ascorbate for maximal activity31.
In situ norepinephrine formation is more complex.
Catecholamines are stored in secretory vesicles; the most thoroughly
investigated are chromaffin vesicles (granules) from the adrenal medulla.
Dopamine beta monooxygenase is exclusively localized to the inside
of the vesicle membrane in soluble and membrane bound forms32,33.
Ascorbic acid is found inside and outside vesicles in mM concentration,
which is as much as 100 fold more than plasma. Surprisingly, ascorbic
acid does not cross the vesicle membrane34. How, then,
is a high concentration achieved in vesicles? Although unknown, the
answer may be that as vesicles are assembled, vitamin C is trapped
inside. Since ascorbic acid is present throughout these cells in high
concentration, newly synthesized vesicles contain a similar high concentration
as found in chromaffin cell cytosol.
In light of this knowledge, it was unclear how vitamin
C was involved in norepinephrine biosynthesis in situ. Since vitamin
C does not have free access to the enzyme that is supposed to use
it, is vitamin C really used by the enzyme in situ? If vitamin C is
used by the enzyme, yet vitamin C cannot cross the vesicle membrane,
what happens when all of the vitamin in the vesicle is consumed? The
solution to these problems is that ascorbic acid within vesicles is
used by the enzyme within vesicles. As ascorbic acid is consumed by
the enzyme, the free radical semidehydroascorbic acid forms within
vesicles35-39. The radical is reduced back to ascorbic
acid by electrons from ascorbic acid on the outside of the granule
membrane, in the cytosol. Electrons from vitamin C in cytosol are
transferred to the protein cytochrome b561 in the granule membrane38,39.
This protein shuttles the electrons across the granule membrane so
that semidehydroascorbic acid inside is reduced. Thus, ascorbic acid
within granules is maintained by ascorbic acid in cytosol, cytochrome
b561, and semihydroascorbic acid35-39. Although cytosol
ascorbic acid is consumed, it is replaced by ascorbic acid transported
from plasma across the cell membrane. The mechanism is displayed in
Figure 3.
Figure 3. Ascorbic acid and catecholamine biosynthesis
in situ. Cytosolic ascorbate transfers an electron to cytochrome b651,
a secretory vesicle transmembrane protein Cytochrome b561 donates
an electron to intravesicular (intragranular) semidehydroascorbic
acid, which forms as intragranular ascorbate reduces dopamine beta-monooxygenase.
Reduced dopamine beta-monooxygenase catalyzes morepinephrine synthesis.
The putative protein responsible for cytosolic accumulation of ascorbate
is indicated by a triangle. To explain the mechanism of electron transfer,
the secretory vesicle is not in proportion to cell size. See text
and references27,35,39 for details.
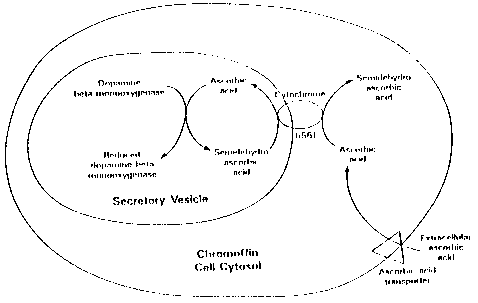
The effect of ascorbic acid on norepinephrine biosynthesis
can be seen from plots of in situ reaction kinetics, or dose response
curves. The first curve is the effect of vitamin C within vesicles
on norepinephrine biosynthesis (Figure 4). New norepinephrine biosynthesis
is displayed on the y axis as a function of internal ascorbic acid,
on the x axis. These data are the first in situ dose response curve
for the effect of an endogenous vitamin on synthesis of a physiologic
substance35. The data indicate that norepinephrine biosynthesis
proceeds at Vmax in situ. This finding is important for
validating in situ kinetics, which proposes that ideal function is
equivalent to maximal synthesis. At least one function should occur
at Vmax in situ; these data demonstrate that this occurs.
The data also show that ascorbic acid is a true co-substrate for norepinephrine
biosynthesis; one ascorbic acid molecule is consumed for each molecule
of norepinephrine formed.
Figure 4. Norepinephrine biosynthesis in chromaffin
granules as a function of intragranular ascorbate concentration. Intragranular
in situ ascorbate concentration is shown on the x-axis; [3H]
norepinephrine biosynthesis is indicated on the y axis. Insets are
Lineweaver-surk and Eadie-Hofstee plots. Reference35 for
details.
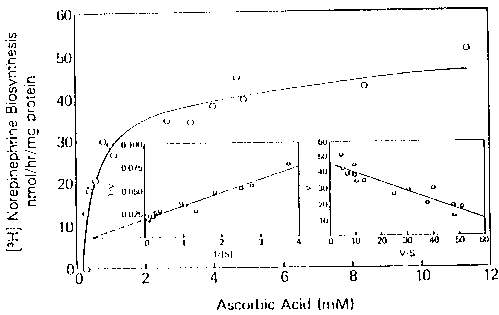
The second dose response curve is the effect of external
vitamin C, in cytosol, on regenerating oxidized vitamin C within vesicles
(Figure 5). The external concentration of vitamin C is shown on the
x axis and regeneration of internal vitamin C is shown on the y axis.
The normal concentration of vitamin C in this cytosol is 5-10 mM.
Thus, regeneration of vitamin C also proceeds at Vmax in
situ36. This finding provides additional support for the
idea that optimal may be equivalent to maximal rate for at least some
reactions which need vitamin C. These data also show that norepinephrine
biosynthesis in situ is different from norepinephrine biosynthesis
for the isolated enzyme. For vitamin requirements, in situ reactions
should be studied.
Figure 5. Regeneration of intragranular ascorbate
as a function of extragranular ascorbate. External ascorbate is on
the x axis; measured intragranular ascorbate is on the y axis. Insets
are Lineweaver-surk and Eadie-Hofstee plots. Reference36
for details.
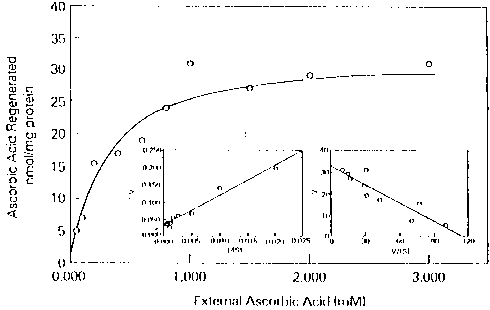
These studies were performed on animal tissue from
adrenal medulla. Although human tissue should show similar characteristics,
these experiments have not been performed because of difficulty in
getting fresh human adrenal tissue. The principles of in situ kinetics
should continue to be formulated using animal tissue, since these
experiments are often easier to perform. However, for applying the
principles of in situ kinetics to vitamin requirements in humans,
the key experiments should be performed using human tissue if possible.
Collagen
biosynthesis in fibroblasts
Ascorbic acid is required by three isolated enzymes
for hydroxylation of procollagen. Hydroxyl groups are inserted on
proline to form 3-hydroxyproline or 4hydroxyproline, and on lysine
to form hydroxylysine40-42. Only when procollagen is hydroxylated
can cross-linking of procollagen occur; cross linking is an essential
part of collagen stability. Ascorbate action on catecholamine biosynthesis
is to hydroxylate a single amino acid derivative. In contrast, for
collagen synthesis ascorbate action is to modify a large polypeptide.
The messenger RNA for this peptide must be transcribed and the message
translated for ascorbate to be effective; ascorbate therefore plays
a role in post-translational modification of procollagen. Ascorbate
may have additional effects on message transcription independent of
a role on post-translational modification43,44.
The action of ascorbic acid has been described for
three enzyme activities for hydroxylation (Table 2). Isolated prolyl
(proline) hydroxylase requires alpha-keto glutarate and ferrous (Fe2+)
iron in addition to ascorbate. The mechanism of hydroxylation may
be via an iron-oxygen complex. Iron is oxidized to Fe3+;
ascorbate may be necessary to reduce iron42,45.
These enzymes are found in fibroblasts, and the effect
of external ascorbate on proline hydroxylation in fibroblasts has
been verified by many investigators (see 42 for review). External
ascorbic acid is added to fibroblasts which have incorporated [3H]
proline. Incorporation of [3H] proline into collagenase-sensitive
protein is measured. In the presence of ascorbate, proline incorporation
is increased several fold.
With respect to vitamin requirements and in situ kinetics,
several key issues remain unknown. One central issue is how much external
and internal ascorbate are required to form specific amounts of hydroxyproline
in fibroblasts. The answer to this issue has clear implications
for collagen biosynthesis in vivo. For example, a high intracellular
concentration of ascorbate might be required for collagen biosynthesis.
To achieve this concentration, ingestion of a certain amount of ascorbate
above the current RDA may be necessary to yield the correct extracellular
vitamin concentration to favor accumulation and subsequent hydroxylation.
Another possibility is that collagen biosynthesis requires only low
intracellular ascorbate concentrations; these concentrations may be
achieved with acorbate ingestion much less than the RDA. In either
ease, the amount of ascorbate which regulates collagen synthesis can
be determined, to give a functional basis to vitamin C requirements.
To determine in situ kinetics for proline hydroxylation,
it is essential to characterize intracellular ascorbate mass and distribution
in normal human fibroblasts as a function of external concentration.
This work is in progress46. It is also necessary to measure
hydroxyproline formation. Previous assays based on [3H]
proline incorporation are indirect and do not measure true hydroxyproline
mass. In addition, unlabelled proline may also be present in cells;
determining the ratio of unlabelled proline to [3H] proline
(specific activity) could be essential for measuring correct hydroxyproline
formation. Thus, direct assays are required to measure proline and
subsequent hydroxyproline for in situ kinetics. A new ultrasensitive
and specific assay for hydroxyproline has recently been developed47.
Using this assay and information about intracellular ascorbate concentrations,
it will be possible to determine in situ kinetics for hydroxyproline
formation in human fibroblasts.
Neutrophils/monocytes
Ascorbic acid was detected in crude fractions of white
blood cells more than 50 years ago, during studies of scurvy in humans48.
Its function, however, is not known. Using oxygen as a starting material,
neutrophils and monocytes generate oxidizing compounds to kill bacteria.
Ascorbate was proposed to be involved in oxidant generation49.
Unfortunately, the evidence is inconclusive. Although neutrophils
form oxidants to kill bacteria, neutrophils must be protected from
these same oxidants. Ascorbate was proposed to be part of the protective
mechanisms, by reducing otherwise toxic oxidants50. Although
an attractive hypothesis, evidence to support it was also inconclusive.
Ascorbate was also proposed to be involved in chemotaxis, or neutrophil
movement. Paradoxically, ascorbate inhibits one of the reactions associated
with chemotaxis, the addition of tyrosine to the microtubule protein
tubulin (tubulin tyrosination)(5).
In situ kinetics in fibroblasts is straightforward
because the function of ascorbic acid is known for isolated proteins.
To apply in situ kinetics in neutrophils, ascorbate function must
be determined first. Some principles of in situ kinetics can be used
to learn ascorbate function. One goal is to be able to measure intracellular
ascorbate concentration and then regulate it across a wide range,
without subjecting animals or humans to scurvy27. Putative
functions, such as oxidant quenching or oxidant generation, could
then be investigated in neutrophils with widely varying intracellular
ascorbate concentrations.
To regulate ascorbate concentration in neutrophils,
we studied ascorbate transport in neutrophils freshly isolated from
normal humans. These neutrophils were 'resting', or not producing
oxidants. Ascorbic acid was accumulated against a concentration gradient
in neutrophils; extracellular ascorbate in micromolar concentration
was accumulated in millimolar concentrations by a high and a low affinity
transport activity. Each was saturable and temperature-dependent52.
Although intracellular ascorbate concentration was
increased by they action of the transport activities, initial endogenous
ascorbate concentration was almost always > 1 mM. To learn ascorbate
function, it was necessary to deplete ascorbate in neutrophils. Unlike
other cell types from which ascorbate is rapidly depleted, ascorbate
was not lost from neutrophils. To deplete ascorbate, we tested whether
substances of similar structure would exchange with intracellular
ascorbate by reverse transport once intracellular saturation occurred.
D-glucose and >-ascorbic acid have a similar ring structure and
chair conformation. We investigated the effects of D-Glucose on ascorbic
acid accumulation and exchange. D-Glucose inhibited the high affinity
activity noncompetitively and the low affinity activity competitively.
Inhibition was completely reversible for each activity. Both transport
activities were almost completely inhibited by glucose concentrations
found in diabetics. Despite competition at the transport sites between
ascorbate and glucose, glucose did not exchange with intracellular
ascorbate, and intracellular ascorbate did not decrease53.
As part of these experiments we made the accidental
discovery that neutrophils making oxidants (activated neutrophils)
accumulated far more ascorbate than the already high concentrations
in resting neutrophils. The mechanism of this accelerated accumulation
is shown in Figure 6. Extracellular ascorbate is oxidized to dehydroascorbic
acid by oxidants from activated neutrophils; dehydroascorbic acid
is preferentially transported and immediately reduced intracellularly
to ascorbate54.
These observations may be a clue to the function of
ascorbate in neutrophils. As shown in Figure 6, extracellular ascorbate
could protect host tissues from oxidant damage by quenching destructive
oxidants. Extracellular ascorbate is oxidized to dehydroascorbic acid.
Preferential transport and immediate reduction to intracellular ascorbate
occurs, with the result that neutrophils contain much more intracellular
ascorbate than they started with. The increase in intracellular ascorbate
occurs at precisely the time when ascorbate is needed to protect against
oxidants, many of which diffuse freely. Thus, extracellular ascorbate
may protect host tissues; oxidized ascorbate is then recycled to protect
neutrophils from their own oxidants.
Figure 6. Antioxidant recycling in human neutrophils:
a model. Extracellular ascorbic acid in micromolar concentration is
oxidized to dehydroascorbic acid by oxidants generated by activated
neutrophils. Dehydroascorbic acid in micromolar or sub-micromolar
concentration preferentially enters neutrophils and is immediately
reduced back to ascorbic acid; the result is accelerated accumulation
of ascorbate. Extracellular ascorbate functions to quench radicals.
Dehydroascorbic acid formed in this fashion is recycled intracellularly
to protect the neutrophil from the oxidants it generates. The proposed
mechanism of reduction is unknown but could require glutathione, NADPH,
and the proteins shown. The mechanism of dehydroascorbic acid entry
is unknown. Abbreviations: AA = ascorbic acid; DHA = dehydroascorbic
acid; GSH = reduced glutathione; GSSG = oxidized glutathione. Reference54
for details.
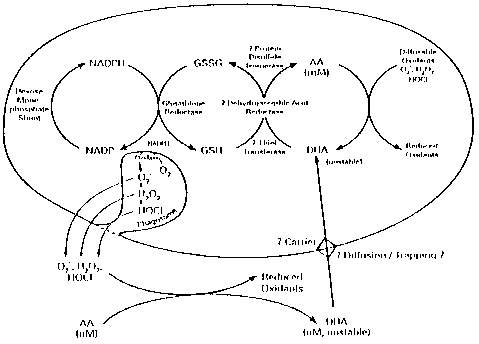
Although oxidant quenching by antioxidant recycling
is an attractive hypothesis, antioxidant recycling (Figure 6) could
have other functions. As some examples, increased intracellular ascorbate
could be used for regulating chemotaxis and/or phagocytosis, or increased
oxidant generation. The last possibility may seem especially attractive
since the isolated enzyme myeloperoxidase, necessary for hypochlorous
acid formation, utilizes ascorbate55. However, the intracellular
distribution of ascorbate is the opposite of that predicted if myeloperoxidase
requires ascorbate in situ52. Myeloperoxidase is found
in neutrophil granules; these granules fuse with the phagosome, and
hypochlorous acid is formed in the phagosome. Ascorbic acid is not
expected in the phagosome for myeloperoxidase to use. Ascorbic acid
in the phagosome would either be transported directly into the cytosol
or oxidized to dehydroascorbic acid and then preferentially transported
into the cytosol. It remains possible that electrons from ascorbate
in cytosol are transferred to myeloperoxidase in phagosomes via electron
transfer proteins38,39. Although monocytes/macrophages
do not have myeloperoxidase, ascorbate function could be any of the
others described above.
Lymphocytes
Since it was known for many years that ascorbic acid
was found in high concentration in crude preparations of white blood
cells, it was assumed the vitamin was accumulated in lymphocytes.
Ascorbic acid accumulation in mM concentration was recently shown
in purified B and T Iymphocytes56. Unlike neutrophils,
ascorbic acid concentrations can be both increased and decreased easily
by culturing lymphocytes56.
The function of ascorbic acid in lymphocytes is unknown.
Ascorbic acid was proposed to regulate antibody production. However,
no consistent effects were found on serum IgG, IgA, or IgM57-58.
There is no clear effect of ascorbic acid on lymphocyte proliferation
in response to mitogens58. There are inconsistent effects
of ascorbate on other parameters such as T helper cell to T suppressor
cell ratios; natural killer functions and mitogen-induced protein
synthesis. Many other mechanisms for ascorbate action have been proposed59,
but none have substantial supporting evidence. As noted by Anderson
and colleagues58, the problem is the lack of a clear biochemical
molecular mechanism for ascorbate action in lymphocytes. The problem
is compounded by conflicting data in animal model systems, species
variations, and non-specificity of some assays used to determine immune
function. Additional complications are due to difficulties in measuring
ascorbic acid and its metabolite, dehydroascorbic acid. The problems
are assay nonspecificity, instability of ascorbate and especially
dehydroascorbic acid, assay interference from other reducing/ oxidizing
substances, and assay insensitivity7.
Since the concentration of ascorbate can be varied
widely in lymphocytes, lymphocyte function can be investigated over
a wide range of intracellular ascorbate concentrations. Which functions
should be investigated? To learn ascorbate's action, specific biochemical/
molecular reactions rather than general lymphocyte function should
be studied. New techniques using molecular genetics should make these
studies possible. Such experiments are now underway in our laboratory.
Carnitine biosynthesis L-Carnitine is a quaternary amino acid
necessary for lipid metabolism. Carnitine acts with two enzymes, carnitine
palmitoyltransferase and carnitine acylcarnitine translocase, to allow
long chain fatty acids to enter mitochondria. Once inside, lipids
are oxidized to generate ATP. Carnitine, in conjunction with the enzymes
carnitine acetyltransferase and carnitine-acylcarnitine translocase,
also facilitates exit of short organic acids from mitochondria, which
may be necessary for efficient mitochondrial function60.
Carnitine is maintained in humans by dietary ingestion
and by endogenous synthesis from lysine and methionine. There are
two hydroxylation steps in carnitine biosynthesis mediated by the
enzymes epsilon-N trimethyllysine hydroxylase and gamma- butyrobetaine
hydroxylase. Both enzymes are monooxygenases which require reduced
iron and ascorbic acid for maximal activity61-63. Thus,
these enzymes are similar to those needed for proline and lysine hydroxylation
discussed above.
While these isolated monooxygenases require ascorbate
for maximal activity, investigation of the requirement in vivo faces
several complex obstacles. This is because the endogenous pool of
carnitine precursors, including substrates for the ascorbate dependent
enzymes, may be difficult to regulate in vivo. Control of these substrate
concentrations may be required to test in situ enzyme activity. Readers
are referred to two thorough excellent reviews of the function of
ascorbic acid in carnitine biosynthesis64,65.
Peptidyl
alpha monooxygenase
Many neuroendocrine peptides and peptide hormones
are synthesized as larger molecules that are then modified before
they become the final active compound. One modification is replacement
of the carboxy terminus with an alpha amidated peptide. For the amidation
to occur, the precursor peptide at the carboxy terminus must be glycine.
Examples of hormones that undergo amidation like this are corticotrophin
releasing hormone, growth hormone releasing hormone, thyrotropin releasing
hormone, oxytocin, vasopressin, gastrin, cholecystokinin, vasoactive
intestinal peptide, calcitonin, secretin, and substance P66.
The enzyme that catalyzes peptide amidation is called
peptidyl alpha monooxygenase (PAM). Similar to dopamine beta-monooxygenase,
PAM is dependent on oxygen, copper, and ascorbate67. In
recent studies PAM has been found to be two enzymes68-70.
The first is peptidyl alpha hydroxylating monooxygenase (PHM), which
requires ascorbate, oxygen, and copper. The second is peptidyl alpha
hydroxyglycine alpha amidating lyase (PAL). PHM and PAL are encoded
together sequentially on a PAM precursor mRNA. The two enzymes remain
intact as a bifunctional protein (PAM) in some tissues such as the
atrium. In other tissues, such as the neuroendocrine pituitary gland,
the protein is cleaved and the two enzymes PAL and PHM are formed.
PAM requires ascorbate for maximal activity in pituitary
tissues. In most other tissues, however, little is known about the
dependence of peptide amidation on ascorbate. The need of PAM and/or
PHM for ascorbate is difficult to study because peptide biosynthesis
involves 1 many steps, many enzymes, and multiple cellular compartments.
PAM and/or PHM act towards the end of peptide biosynthesis. Regulation
of substrate concentration at these steps is not straightforward.
Nevertheless, since small alpha amidated peptides may have many physiologic
roles, regulation of their biosynthesis should be understood. These
issues have been reviewed in detail by the leaders in this field70.
Summary
Ascorbic acid is required for at least eight intracellular
enzymatic reactions, and for other intracellular nonenzymatic reactions.
Extracellular reactions may also be dependent on ascorbate as an electron
donor. Many of these reactions can be studied in relation to ascorbate
concentration using the principles of in situ kinetics. By understanding
vitamin C dependent reactions in situ as a function of vitamin C concentration
in situ, vitamin requirements can be developed with a biochemical
and functional basis.
References
- Hodges RE, Baker EM, Hood J, Sauberlich HE, March
SC. Experimental scurvy in man. Am J Clin Nutr 1969; 22:535-48.
- Hodges RE, Hood J, Canham HE, Sauberlich HE, Baker
EM. Clinical manifestations of ascorbic acid deficiency in man.
Am J Clin Nutr 1971; 24:432-43.
- Baker EM, Hodges RE, Hood J, Sauberlich HE, March
SC. Metabolism of ascorbic 1-'4C acid in experimental human scurvy.
Am J Clin Nutr 1969; 23:905-12.
- Baker EM, Hodges RE, Hood J, Sauberlich HE, March
SC, Canham JE. Metabolism of 14C and 3H labelled Lascorbic acid
in human scurvy. Am J Clin Nutr 1971; 24:1~1 54.
- Recommended dietary allowances. Subcommittee on
the 10th edition of the RDAs, food and nutrition board commission
on life sciences, national research council, 10th edition. Washington
D.C.: National Academy Press; 1989: pp 115-24.
- Kallner A, Hartman D, Hornig D. Steady-state turnover
and body pool of ascorbic acid in man. Am J Clin Nutr 1979; 32:530-39.
- Washko PW, Welch RW, Dhariwal KR, Wang Y, Levine
M. Ascorbic acid and dehydroascorbic acid analysis in biological
samples. Analytical Biochemistry, 1992; 204:114.
- Jacob RA, Pianalto FS, Agee RE. Cellular ascorbate
depletion in healthy men. J Nutr 1992; 122:1111-1118.
- Levine M. N Eng J Med 1986; 314:892-902.
- Eaton SB, Konner M. Paleolithic nutrition. N Eng
J Med 1985; 312:283-9.
- Levine M, Cantilena CC, Dhariwal KR. In situ kinetics
and ascorbic acid requirements. World Rev Nutr Diet 1993; in press.
- Stein HB, Hasan A, Fox IH. Ascorbic acid induced
uricosuria. Ann Int Med 1976; 84:385-8.
- Balcke P, Schmidt P, Zazgornik J, Kopsa H, Haubenstock
A. Ascorbic acid aggravates secondary hyperoxalemia in patients
on chronic hemodialysis. Ann Int Med 1984; 101 :344-6.
- Piesse JW. Nutritional factors in calcium containing
kidney stones with particular emphasis on vitamin C. Int Clin Nutr
Rev 1985; 5:110-129.
- Rivers J. Safety of high level vitamin C ingestion.
Ann NY Acad Sci. 1987; 498:445-53.
- Riemersma RA, Wood DA, Macintyre CC, Elton RA,
Gey KF, Oliver MF. Risk of angina pectoris and plasma concentrations
of vitamins A, C, and E and carotene. 42 Lancet 1991; 337 (8372):1-5.
- Gey KF, Puska P, Jordan P, Moser UK. Inverse correlation
between plasma vitamin E and mortality from ischemic heart disease
in cross-cultural epidemiology. Am J Clin Nutr 1991; 53:326S-34S.77.
- Simon JA. Vitamin C and cardiovascular disease:
a review. J Am Coll Nutr 1992; 11:107-25.
- Enstrom JE, Kanim LE, Klein MA. Vitamin C intake
and mortality among a sample of the U . S. population. Epidemiology
1992; 3:194-202.
- Steinmetz KA, Potter JD. Vegetables fruit and cancer
II: mechanisms. Cancer causes and control 1991; 2:427-42.
- Byers T, Perry G. Dietary carotenes, vitamin C,
and vitamin E as protective antioxidants in human cancers. Annual
Review of Nutrition 1992; 12:139-59.
- Ziegler RG. Vegetables, fruits, and carotenoids
and the risk of cancer. Am J Clin Nutr 1991; 53:251s-259s.
- Ziegler RG, Brinton LA, Hamman RF. Diet and the
risk of invasive cervical cancer among white women in the United
States. Am J Epidemiol 1990; 132:432-45.
- Robertson JM, Donner AP, Trevithick JR. A possible
role for vitamins C and E in cataract prevention. Am J Clin Nutr
1991; 53:346-51S.
- Jacques PF, Chylack LT. Epidemiologic evidence
of a role for the antioxidant vitamins and carotenoids in cataract
prevention. Am J Clin Nutr 1991; 53:352-5.
- Levine M, Hartzell WO. Ascorbic acid: the concept
of optimum requirements. Ann NY Acad Sci 1987; 498:424-44.
- Levine M, Dhariwal KR, Washko P, Wang Y-H, Welch
R, Bergsten P, and Butler EJ. Ascorbic acid and in situ kinetics:
a new approach to vitamin requirements. Amer J Clin Nutr 1991; 54:1157S-1162S.
- Lewin S. In: Vitamin C: its molecular biology and
medical potential. New York: Academic Press, 1976: 5-39.
- Stadtman ER. Ascorbic acid and oxidative inactivation
of proteins. Am J Clin Nutr 1991; 54:1125S-28S.
- Meister A. On the antioxidant effects of ascorbic
acid and glutathione. Biochem Pharm 1992; 44:1905-15.
- Friedman S, Kaufman S. J Biol Chem 1965; 240:4763-73.
- Belpaire F, Laduron PL. Tissue fractionation and
catecholamines. Biochem Pharmacol 1968, 17:411-21.
- Laduron PL. Evidence for localization of dopamine
betahydroxylase in within the chromaffin granules. FEBS Lett 1975;
52:132-34.
- Tirrell JG, Westhead EW. The uptake of ascorbic
acid and dehydroascorbic acid by chromaffin granules of the adrenal
medulla. Neuroscience 1979; 4:181-6.
- Dhariwal KR, Washko P, Hartzell WO, Levine M. Ascorbic
acid within chromaffin granules: in situ kinetics of norepinephrine
biosynthesis. J Biol Chem 1989; 264:15404-409.
- Dhariwal K, Shirvan M, Levine M. Ascorbic acid
regeneration within chromaffin secretory vesicles: in situ kinetics.
J Biol Chem 1991; 266:5384-87.
- Dhariwal KR, Black CDV, Levine M. Semidehydroascorbic
acid as an intermediate in norepinephrine biosynthesis in chromaffin
granules. J Biol Chem 1991; 266: 12908-914.
- Fleming PF, Kent UM. Cytochrome b561, ascorbic
acid and transmembrane electron transfer. Am J Clin Nutr 1991; 54:1173S-1178S.
- Njus D, Jalukar V, Zu J, Kelley PM. Concerted protonelectron
transfer between ascorbic acid and cytochrome b561. Am J Clin Nutr
1991; 54:1179S-83S.
- Prockop DJ, Kivrikko KI, Tuderman L, Guzman NA.
The biosynthesis of collagen and its disorders (two parts). N Eng
J Med 1979; 301:13-23;77-85.
- Kivirikko KO, Myllyla R, Pihlajaniemi T. Protein
hydroxylation: prolyl4-hydroxylase, an enzyme with four cosubstrates
and a multifunctional subunit. FASEB J 1989; 1609-17.
- Peterkofsky B. Ascorbate requirement for hydroxylation
and secretion of procollagen: relationship to inhibition of collagen
synthesis in scurvy. Am J Clin Nutr 1991 54:1135S-40S.
- Chojkier M, Houghlum K, Solis-Herruzo JA, Brenner
DA. Stimulation of collagen gene expression by ascorbic acid in
cultured human fibroblasts. A role for lipid peroxidation? J Biol
Chem 1989; 264:16957-62.
- Houglum KP, Brenner DA, Chojkier M. Ascorbic acid
stimulation collagen biosynthesis independent of hydroxylation.
Am J Clin Nutr 1991; 54:1141S-43S.
- Haunauske-Abel HM, Gunzler V. A stereochemical
concept for the catalytic mechanism of prolyl hydroxylase: applicability
to classification and design of inhibitors. J Theor Biol 1982; 94:421-55.
- Butler JDB, Bergsten P, Welch RW, Levine M. Ascorbic
acid accumulation in human skin fibroblasts. Am J Clin Nutr 1991;
54:1144S-46S.
- Welch R, Ackworth I, Levme M. Coulometric electrochemical
detection of hydroxyproline using 7-chloro-4-nitrobenzo-2-oxa-1,3-diazole.
Analyt Biochem 1993; 210:199-205.
- Crandon JG, Lund CC, Dill DB. Experimental human
scurvy. N Eng J Med 1940; 223:353-69.
- Thomas EL, Learn DB, Jefferson MM, Weatherred W.
Superoxide-dependent oxidation of extracellular reducing agents
by isolated neutrophils. J Biol Chem 1988; 263:2178-86.
- Halliwell B, Wasil M, Grootveld M. Biologically
significant scavenging of the myeloperoxidase-derived oxidant hypochlorous
acid by ascorbic acid: implications for antioxidant protection in
the inflamed rheumatoid joint. FEBS Lett 1987; 213:15-17.
- Nath J, Gallin. Effect of vitamin C on tubulin
tyrosinolation in polymorphonuclear leukocytes Ann NY Acad Sci 1987;
498:216-228.
- Washko P, Tortosen D, Levine M. Ascorbic acid transport
and accumulation in human neutrophils. J Biol Chem 1989; 264:18996-19002.
- Washko PE, Levine M. Inhibition of ascorbic acid
transport in human neutrophils by glucose. J Biol Chem 1992; 267:23568-574.
- Washko PW, Wang Y, Levine M. Ascorbic acid recycling
in human neutrophils. J Biol Chem 1993; In press.
- Marques LA, Dunford HB, Van Wart H. Kinetic studies
on the reaction of compound II of myeloperoxidase with ascorbic
acid. J Biol Chem 1990, 265:5666-5670.
- Bergsten P, Amitai G, Kehrl J, Dhariwal K, Klein
H, Levine M. Millimolar concentrations of vitamin C in purified
mononuclear leukocytes: depletion and reaccumulation. J Biol Chem
1990; 265:2584-2587.
- Anderson R, Oosthuizen R, Maritz R, Theron A, Van
Rensburg AJ. The effects of increasing weekly doses of ascorbate
on certain cellular and humoral immune functions in normal volunteers.
Am J Clin Nutr 1980 33:71-76.
- Anderson R, Smit MJ, Joone GK, Van Staden AM. Vitamin
C and cellular immune functions. Ann NY Acad Sci 1990; 587:34-38.
- Bendich A. Antioxidant vitamins and their functions
in immune responses. Adv Exp Med Biol 1990; 262:35-55.
- Feller AG, Rudman D. Role of carnitine in human
nutrition, 1988; 118:541-7.
- Hulse JD, Ellis SR, Henderson LM. Beta-Hydroxylation
of trimethyllysine by an alpha-ketoglutarate-dependent mitochondrial
dioxygenase. J Biol Chem 1978; 253:1654-9.
- Lindstedt G, Lindstedt S. Co-factor requirements
of gamma-butyrobetaine hydroxylase from rat liver. J Biol Chem 1970;
245:4178-86.
- Dunn WE, Rettura G, Seifter E, Englard S. Carnitine
biosynthesis from gamma-butyrobetaine and from exogenous protein-bound
6-N-trimethyl-L-lysine by the perfused guinea pig liver. J Biol
Chem 1984; 259: 1076~70.
- Rebouche CJ. Ascorbic acid and carnitine biosynthesis.
Am J Clin Nutr 1991; 54:1147S-52S.
- Englard S, Seifter S. The biochemical functions
of ascorbic acid. Ann Rev Nutr 1986; 6:365 406.
- Wand GS, Ney RL, Baylin S, Eipper B, Mains RE.
Characterization of a peptide alpha-amidation activity in human
plasma and tissues. Metabolism 1985; 34:1044-51.
- Murthy ASN, Mains RE, Eipper BA. Purification and
characterization of peptidylglycine alpha-amidating monooxygenase
from bovine neurointermediate pituitary. J Biol Chem 1986; 261:1815-22.
- Stoffers DA, Ouafik LH, Eipper BA. Characterisation
of novel mRNAS encoding enzymes involved in peptide alpha-amidation.
J Biol Chem 1991; 266:1701-7.
- Eipper BA, Perkins SN, Husten EJ, Johnson RC Keutmann
HT, Mains RE. Peptidyl-alpha-hydroxyglycine alpha-amidating lyase
purification, characterization and expression. J Biol Chem 1991;
7827-33.
- Eipper BA, Mains RE. The role of ascorbate in the
biosynthesis of neuroendocrine peptides. Am J Clin Nutr 1991; 54:1153S-56S.
Copyright © 1993 [Asia Pacific Journal of Clinical
Nutrition]. All rights reserved.
to the
top