INVITED REVIEW
ARTICLE
Food memory: neuronal involvement
in food recognition
Asia Pacific J Clin Nutr (1992)1(1): 3-12
Hisao Nishijo and Taketoshi Ono
Department of Physiology, Faculty of Medicine,
Toyama Medical and Pharmaceutical University, Toyama , Japan.
Previous studies indicate that ablation of the temporal
cortex including the amygdala (AM) and hippocampal formation (HF) induce the Kluver-Bucy syndrome in which animals
cannot discriminate food from nonfood. Of 710 AM neurons tested, 129
(18.2%) responded to single sensory stimulation (48 to vision, 32 to audition,
49 to ingestion), 142 (20.0%) to multimodal
stimulation and 20 to only one item with affective
significance. Eight food related AM neurons
were tested in reversal by salting food or introducing
saline, and all responses were modulated by reversal. In HF and parahippocampal cortices (PH), 864
neurons were recorded, and 160 (18.5%) responded lo the sight of certain objects.
Of these, 23 responded predominantly to food related rewarding objects,
13 to several aversive objects such as a spider model, syringe,
objects associated with weak electric shock, ten to one object or one
kind of object, seven to unfamiliar objects. Of 14 rewarding or aversive object-related neurons
tested, responses of seven
to the same test object did not change in extinction or reversal tests.
Although responses of the other seven decreased in extinction or
reversal tests, the magnitude of response remaining in five of those seven still exceeded that of
responses to other categories. Results suggest complementary AM and HF-PH functions. The AM may be important in ongoing
recognition of the affective significance of complex stimuli (food-nonfood discrimination)
and the HF-PH in
sustaining past affective significance
Introduction
The mammalian limbic system and hypothalamus are
important interacting structures in the control
of functions such as feeding behavior3,11,33.The limbic
system affects the behaviour of an organism mainly through
parts of the brainstem and diencephalon such as the hypothalamus8. The amygdala (AM), the
hippocampal formation (HF) and parahippocampal cortices (PH) are the principal limbic regions, in which various
exteroceptive sensations converge6,14.Various
exteroceptive information may reach the lateral hypothalamic area (LHA), feeding center and
ventromedial hypothalamic nucleus (VMH), satiety
center through the AM and HF that send efferent fibers to these two centers22,27,28 Lesion
studies indicate some contributions of both the AM and HF to learning and recognition of rewarding objects such as food
in some situations8.
For example, the animals with bilateral hippocampal lesions had deficits in forming association of conditioned stimuli with unconditioned stimuli,
ie reward, if delay time was introduced between conditioned and unconditioned
stimuli8. Bilateral lesions of the temporal cortices including the AM produce the
Klaver-Bucy syndrome7,13 All animals with these deficits excessively examined
objects whether food or nonfood, by touching, tasting and smelling. These animals
seem to be indifferent to the reward value of a stimulus12.
We have been investigating neuronal responses in rat
and monkey AM, HF and LHA during discrimination
of rewarding and aversive stimuli, such as food and nonfood. A series of our experiments reveal functional
roles of each of these areas, and indicate sequential visual information processing for recognition
of a rewarding object, ie food,20,23. We review here our recent results in monkey experiments18,19,37.
Experimental setup and data analysis
Eight monkeys (Macacafuscata, 4-6 kg) were
studied. Each was restrained painlessly in a stereotaxic apparatus
by a previously prepared surgically fixed head holder designed in our laboratory24,25for
single unit recording. They sat in a chair facing a panel containing
shutters and an operant response bar (Fig. lA).
Juice, water, and saline were made available through
a small spout with an electromagnetic valve. Aversive stimulation was by a weak
electric shock applied between the ear lobes. The task included visual discrimination
(feeding, drinking, and active avoidance), and
auditory discrimination, which led to presentation of food
or nonfood, or various sensory stimuli associated with food,
juice or water (Fig. IB, C)18,19.
During a recording session behaviour and eye movement were monitored
by TV cameras or electrooculography,
or both, as well as by direct observation by the experimenter.
Two task situations, an original one and a modified
version of this, were used. In the original
version of the feeding task an opaque shutter (Wl) was opened
at random intervals to reveal a transparent shutter (W2) in front
of a stage. By pressing the bar a pre-determined number of times (fixed
ratio, FR 10-30) monkeys could take an object seen through W2 and
in the case of desirable food ingest it (Fig. 1B, solid line). Similarly,
when the FR criterion was met in a drinking task, W1 was automatically
closed and a drop of potable liquid such as juice or water, portended
by a symbolic object such as a column or cube could be licked from
a small spout (Fig. 1B, dashed line); eg a white cylinder was usually
associated with juice and a red cylinder was associated with water.
In active avoidance tasks a brown cylinder was usually associated
with a weak electric shock. On seeing the brown cylinder and hearing
a 1200 Hz tone monkeys had to complete a FR schedule within 4 6 seconds
to avoid electric shock. The required bar press procedure closed W1
without producing the shock (Fig. 1B, dashed line). In the auditory
discrimination task a buzzer noise was associated with food, ie cookies
or raisins, and a tone with a fundamental frequency of 800
Hz was associated with a drop of juice (Fig. IC). On hearing the buzzer
or the 800 Hz tone the monkey had to complete a FR schedule to obtain
the corresponding food or juice, as in the feeding task. Two pure
tones (2800 or 4300 Hz) were introduced as neutral stimuli associated
with neither reward nor electric shock and the food or test objects
not being visible behind the shutter W1.
 |
|
Figure 1.
Schema of an experimental setup (A) and time sequences of two operant
tasks (B,C). A: monkey sat in a chair facing a
panel with a bar, and a window covered by two shutters (W1, an opaque
shutter; W2, a transparent shutter) in front of a stage. Liquid could
be obtained from a spout near the monkey's mouth. B: time sequence
of visual discrimination tasks that involved feeding (------------),
drinking (- - -), or avoidance (- - -). W1 and W2 opened at Up. C:
time sequence of auditory discrimination task. Tone started at On.
BP: indications of individual bar presses and time during which they
occurred (B, C). Liquid was dispensed from spout after last bar press
if a particular object was presented. Tone indicated availability
of reward in auditory discrimination test (C). Reward: drop of juice
dispensed from spout after last bar press, or cookie or raisin on
stage became available by simultaneous opening of W1 and W2 after
last bar press.
In a modified version of the task the monkey could
see an object on a stage through a one-way mirror
(S1) in front of the stage when a light behind the mirror was turned on. However, a second shutter (S2) prevented
access to the bar. After a delay of at least 2 seconds, S2
was opened automatically so the bar could be
pressed. The Sl shutter was opened by the last bar press. The monkey's behaviour and the neuronal responses
were essentially the same in this and the previously described
situation. The task comprised three phases (Fig.
1C): (1) control; (2) discrimination visual or auditory discrimination of
an object or situation; (3) operant responding (bar pressing), and
(4) ingestion or avoidance.
We focused on possible discharge patterns of single
AM, HF or PH neurons when these different rewarding
(food) and aversive stimuli were presented. Some neurons were tested
by changing the affective (rewarding or aversive) significance of a stimulus.
Amygdalar neuron responses
Of 710 AM neurons tested, 380 responded to at least
one stimulus. Based on their responsiveness
to sensory modalities, 291 of these 380 neurons fell into one of five categories: vision-related, audition-related,
ingestion-related, multimodal and selective.
Forty-eight neurons responded, all positively to visual
stimuli, but not to auditory, oral sensory or
somesthetic stimuli. These neurons responded clearly to the sight
of unfamiliar objects, regardless of whether they were food or nonfood, and to the sight of some familiar
nonfood objects. Of 48
vision-related neurons, 31--Vis-I type --responded
consistently both to rewarding objects, such
as familiar food and red or white cylinders associated with water
or juice, and to certain nonfood items such as a brown cylinder associated with electric shock, or a syringe, glove or other object, but not to
familiar neutral nonfood stimuli, ie tape. The remaining 17 vision related neurons--Vis-II
type--responded similarly to unfamiliar objects and to certain familiar negative
objects, but not to familiar reward-related objects, ie foods
and red and white cylinders associated with
water and juice. Figure 2 illustrates responses of a typical
Vis-I neuron. This neuron responded strongly to both familiar positive (Aa, orange) and negative (Ab, brown
cylinder) objects, but not to familiar neutral tape (Ac, tape). In contrast to its responses to visual stimuli, this
neuron did not respond to any auditory stimuli. An example of no response to a familiar positive auditory stimulus
is shown in Fig. 2Ad. Those stimuli did, however, elicit various overt reactions, such as bar pressing.
Figure 2Aa shows no response
after putting orange into the mouth (indicated by arrow), so this neuron did
not respond to oral sensory stimuli. Responses of this particular
neuron to various food and nonfood objects are compared in Fig. 2B.
The degree of preference for different kinds of food was estimated from evaluation of
the animal's overt behavior
when the different food items were
made available to it. The neuron responded significantly more strongly
to more preferred food than to less
preferred food.
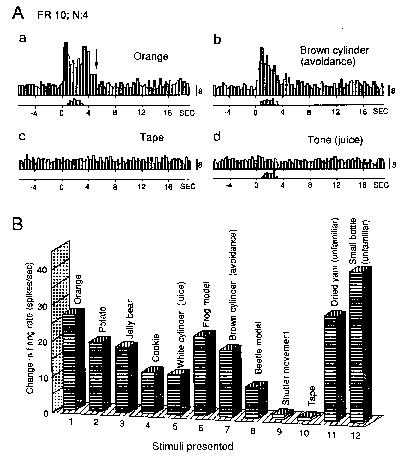 |
Figure 2.
Responses of Vis-I AM neuron. A: activity increased
at the sight of positive (a) and negative (b) affect-related, but
not neutral, objects (c). However, neuron did not respond to positive
affect-related auditory stimuli (800 Hz fundamental frequency tone)
(d). Note absence of neuronal response after animal put orange into
mouth (arrow in Aa). Bar presses shown by histograms on time scales
(W1 opened at time 0). Responses are shown as the sum of 4 trials.
Calibration at right of each histogram: number of spikes in each bin.
B: Comparison of Vis-I neuron responses to various objects. Each column
rearranged here to compare among different foods (columns 1-5), various
nonfood objects (columns 6-8) neutral (columns 9, 10), and unfamiliar
objects (columns 11, 12) shows response magnitude after the indicated
objects were revealed, ie the mean discharge rate (spikes/sec) of
four 5-sec firing rates minus spontaneous rate. Unfamiliar stimuli
evoked the greatest responses. Neuronal responses to objects shown
here (and to other objects not shown) correlated with rates of behavioural
responses.The response profile of Vis-I neurons indicates that although
the response magnitude may vary, these neurons respond to biologically
significant objects regardless of whether they can be considered rewarding
or aversive. To investigate this inference, the neuron shown in Fig.
2 was also studied in various situations in which affective significance
of the stimulus was altered (Fig. 3). Responses to an unfamiliar object,
dried yam which the monkey apparently did not consider to be food,
habituated gradually in trials 1 to 14 as the object became familiar
and meaningless. In trials 13 and 14 the monkey never pressed the
bar (not shown), which indicates that it learned that the object was
biologically meaningless. When the dried yam plus the 1200 Hz tone
was presented instead of the brown cylinder plus the 1200 Hz tone
(avoidance task) without electric shock, the initial response was
slight, and quickly habituated in trials 15 to 18 as the monkey learned
that the dried yam was still meaningless. In trial 19 the dried yam
plus the tone was followed by electric shock. In trial 20 the neuronal
response was elicited without bar pressing for avoidance. In trials
21 and 22 both neuronal and behavioural responses were elicited. The
bar press data are not shown here. Thus, the neuronal response to
the sight of dried yam was modified when associated with other stimuli
(1200 Hz tone and electric shock) evincing overt avoidance behaviour.
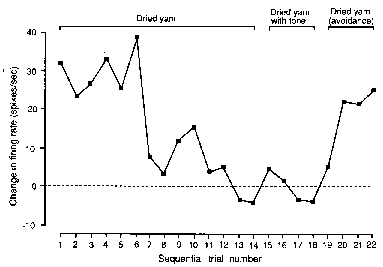 |
Figure 3. Modulation
of Vis-I neuron responses to a new food,
dried yam. The dried yam, which was handled and smelled but never
tasted, finally was not accepted by the monkey as food. In trials
1-14 the response gradually habituated. In trials 15-18 the dried
yam was presented with the 1200 Hz tone used in the active avoidance
task. In trials 19-22 the response appeared again after association
with electric shock (in trials 19 and 20 electric shock was applied).
Shock was avoided in trials 21 and 22. ·[squares] indicate mean response
magnitude (net response minus spontaneous firing rate) during 5 sec
presentation of the dried yam.
Thirty-two neurons responded exclusively to auditory
stimuli with the same responsiveness to affective significance as vision-related neurons. All of
these 32 neurons responded vigorously to unfamiliar sounds and habituated to certain auditory stimuli in repeated
trials; none responded to familiar pure tones used as controls. These neurons were subdivided into two groups:
11 that responded to familiar cue tones associated with a cookie or juice reward, as well as to unfamiliar sounds
(aud-I); and 21 that responded to unfamiliar sounds but not to cue tones associated with cookie or juice (aud-II).
Fortynine neurons responded primarily during the ingestion
phase of the tasks. According to responsiveness
to other stimuli, this group was subdivided into three
groups: 32 oral sensory, 13 oral sensory plus vision, and four oral sensory plus audition. The responses of 142 neurons
were multimodal. Of these,48 responded physically and nonspecifically to various sensory stimuli. These
responses were independent of the nature of the stimuli.
In contrast to the phasic type neurons, 94 neurons
responded tonically to biologically significant stimuli, but not to familiar neutral stimuli.
Responses of 20 neurons were highly selective for
only particular familiar objects or sounds:
nine were selective for one specific food item or for cylinders associated with potables, eight were selective
for one specific nonfood item, and three were selective for one specific sound. Examples of responses that indicated
selectivity for one specific food item are shown in Fig. 4. This neuron was tested with 16 objects and
seven somesthetic and
auditory stimuli (not all shown), and the magnitude of its responses to any other stimulus relatively insignificant (Fig. 4A). The responses
of this neuron to the sight of watermelon were also modulated by the affective significance (Fig. 4B). This
neuron responded consistently to the sight of watermelon in trials
1 and 2. In trials 7-11, after four intervening
trials, salted watermelon reversibly modified the neuronal
activity. The salted watermelon was visually indistinguishable from
unsalted watermelon and in trial 7 the neuron
responded as previously. However, as soon as
the salted watermelon was ingested, the activity
suddenly decreased (not shown). In trials 8- 11 the response
to the sight of watermelon rapidly decreased
and finally disappeared.
In trials 8 and 9 bar pressing failed to meet
the FR criterion to obtain the watermelon and
in trials 10 and 11 bar pressing stopped entirely (not shown).
After the experimenter gave a piece of unsalted
watermelon to the animal by hand, neuronal and bar pressing
responses (not shown) resumed in trials 12 and 13.
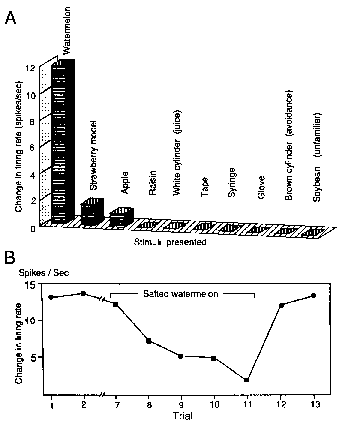 |
|
Figure 4. Responses
of selective AM neuron. A: responses of neuron selective to watermelon.
Other descriptions as for Fig. 2B. B: Modulation of responses to
watermelon by salting. Neuron responded to sight and ingestion of
normal watermelon in trials 1 and 2. Note neuronal response to watermelon
decreased after the trial 7 in which the the animal put salted watermelon
into its mouth. In trials 8-11 the neuronal responses gradually decreased
and finally disappeared. Other descriptions as for Fig. 3.
The responses of eight rewarding-stimuli-related
neurons (four oral sensory plus vision and four selective) and their
related behavioral responses were suppressed in the same way in reversal
tests. During the tests neuronal and integrated behavioral responses
not individual bar presses, were well correlated. The responses of both types
of neurons were first suppressed during the initial ingestion of salted food, suppression
of visual responses then followed although appearance
of the food was not changed. In preliminary
experiments, we observed suppression of gustatory responses
by quinine, and those results were similar to the suppression
by salt that is reported here. The gustation
phase of ingestion is important in the evaluation of food
palatability2,21. The evidence suggests that the suppression
of visual responses by ingestion, as discussed
here, is related to aversion to the taste of salted food.
This speculation is reasonable
since behavioural responses were also suppressed after the first of a series of
salted food trials. This dependence of visual responses on sensation during ingestion suggests that these neurons were
related to visual-oral sensory, possibly gustatory, association18,19.
Geschwind5 suggested that visual gustatory association in the limbic system was essential
for stimulus-reinforcement association. Such associations may
partially account for the deficits in discrimination
of food and nonfood that are observed in the Kluver-Bucy
syndrome caused by lesion of the temporal cortex, including the amygdala
(AM)13. The AM may be important in ongoing recognition
of the affective significance of complex stimuli involved in food-nonfood discrimination.
Some vision-related, audition-related, and multimodal
tonic neurons responded equally to rewarding
and aversive stimuli, but did not respond to familiar neutral
stimuli. The responses of those neurons were
easily modulated by extinction or by changing the affective significance of the stimulus (Fig. 3). Neurons
with those characteristics may discriminate stimuli that are biologically significant
from stimuli that are nonsignificant. This rapid and flexible change
could be a neurophysiological basis of the role of the basolateral amygdala in acquisition of fear-potentiated startle,
depending on the affective significance of the stimulus15.
Those response changes may also be part of attention, reflected by the animal's concentration on one
biologically significant stimulus among various exteroceptive
stimuli18,19,23,26.
Responses of HF and PH neurons
Of 864 neurons recorded during performance of an
operant task coupled to the presentation of
rewarding, aversive or unfamiliar objects, 160 (18.5%) responded to the sight of certain object(s)37.
Of these 160, 73 (61 excited;
12 inhibited) responded to virtually all objects with no significant difference in response magnitude
(nondifferential neurons). Eighty-seven neurons
(66 excited, 11 inhibited, ten excited or inhibited depending
on the kind of objects) responded differentially to different objects
with significant differences in response magnitude or direction -"differential' neurons.
Of these 87 differential neurons, 23 responded significantly
more to rewarding objects than to aversive objects or to unfamiliar objects -rewarding-object-dominant
neurons. An example of this type of neuron is shown in Fig. 5A. Responses to rewarding objects (apple,
raisin, cookie, and a red cylinder associated with juice) were stronger than those to aversive objects (syringe,
spider model, centipede model, white cylinder associated with shock) or those to unfamiliar objects (car model,
clip). However, the order of magnitude of responses to rewarding objects (food)
did not necessarily correspond to the order of the animal's preference
for the objects. Thirteen neurons responded more to aversive objects than
to rewarding objects or unfamiliar objects aversive-object dominant
neurons. Figure 5B shows an example of aversive-object-dominant
neuron responses. The magnitudes
of responses to aversive objects (syringe, spider model, centipede model and white cylinder associated with shock)
were significantly larger than those to objects in the rewarding or unfamiliar groups.
Seven differential neurons responded significantly more to unfamiliar
than to familiar objects unfamiliar-object-dominant neurons.
Ten differential neurons each responded strongly to only one object or to one category of object.
Fourteen rewarding-object and aversive-object dominant
neurons were tested in extinction or reversal tests. Responses of seven neurons to the same
test objects did not change in extinction or reversal tests. Although
responses of the other seven neurons decreased in extinction or reversal tests, the remaining
magnitude of responses of five of these seven still exceeded the magnitudes of their responses to objects in other
categories. Figure 5C shows the data of an aversive-object dominant
neuron that responded strongly during reversal learning to the white
cylinder associated with electric shock. In reversal learning, a white cylinder
usually associated with electric shock was changed to be associated with juice
reward. Though the response magnitude weakened slightly in the last
five trials, this neuron continued to respond with significantly greater
magnitude to the white cylinder than to the red cylinder, which
elicited the greatest magnitude of response
of all of the normal reward-related stimuli (Fig. 5B).
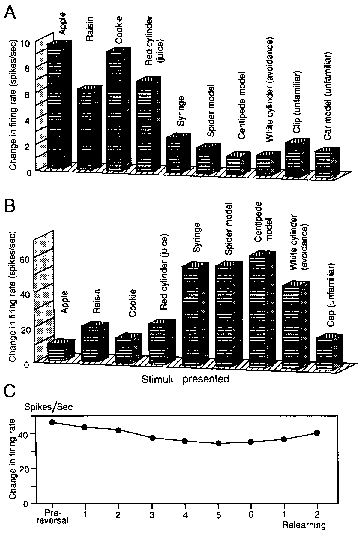 |
Figure 5.
Responses of HF and PH neurons to various food and nonfood objects. A: Responses of rewarding-object-dominant neuron. B:
Responses of aversive-object-dominant neuron.
Each column indicates response magnitude (mean of 1.0 sec firing rate after visual
stimulation minus 1.0 sec firing rate before visual
stimulation). C: Responses of an aversive-object-dominant
neuron in the HF to white cylinder that was usually associated
with electric shock
in pre-reversal block, each reversal block, and two relearning blocks. Each response
indicated by a filled circle shows a mean response
size of five trials. |
The absence of modulation of the differential responses
of HF and PH neurons by reversal or extinction was in contrast to the action of AM neurons. This
is consistent with the
lesion study by Jones and Mishkin'° in which HF lesion had no effect on the performance
of monkeys in stimulus-reward
association tasks. The results suggest that activity of these neurons
might not be directly related to ongoing recognition of affective significance, but
might be related to past memory of affective significance. This agrees
with human studies in which lesion of ventromedial parts of the temporal
cortex including the HF resulted in retrograde amnesia for
a few years36. This part of memory might be related to a
kind of temporal buffer memory30
before being encoded into long-term memory. Neurophysiological and anatomical studies suggest that activity of these
neurons might be involved in the control of feeding behavior. Neurophysiologically, the HF has profound influence
on the hypothalamic ventromedial nucleus and
the perifornical area14 partially by way
of AM projections to the hypothalamus27. A recent anatomical study indicates
direct reciprocal connections between the AM and the HF indicating that the AM and HF may work
in coordination. Thus
the HF may transfer some past affective significance of an object
to the AM for ongoing recognition of food and nonfood.
Neural mechanisms for discrimination
of food and nonfood
Although AM-lesioned animals cannot discriminate
food from nonfood immediately after the operation,
they eventually reacquire the ability to discriminate food9. Furthermore, AM-lesioned animals can learn visual
discrimination7,29,32,39 ,albeit gradually35,38.
Discrimination of food and nonfood can depend on simple visual discrimination38.
It has been suggested that the temporal stem38 or direct
visual projection from the visual cortex to the striatum16 is responsible for such simple discrimination.
Mishkin and his colleagues16,35 suggested that this
inferotemporal-striatal system corresponds to
a neural counterpart of habit or procedural memory4, whereas the
inferotemporal-AM system is responsible for
associative memory by which animals discriminate positive and negative affective objects. AM-lesioned animals
may plastically substitute the inferotemporal-striatal system
for the ITCx-AM system. Food-responsive neurons
have been reported in
the rostroventral putamen17, an area that
receives afferents from both the ITCx and the
AM31,34. In contrast to the responses of AM food-responsive
neurons, responses of the putamen neurons were highly task- dependent and not multimodal, ie they did not
respond during the ingestion
phase. These characteristics suggest strong relations of such neurons
to a procedural memory systeml7,23.
The ratio of selective neurons found in the AM and
HF to the total number of tested, 30/1574, appears
to be very small in relation to the total number of items or
objects with which the animals were familiar. However, if we consider that the number of items used to
test each neuron was probably insignificant compared to the total admittedly relatively limited experience of laboratory
animals the activity of selective neurons remained very low when tested with any other than the selective
item.
Thus we can draw two conclusions: (1) the number of
selective neurons identified was very small
compared to the unknown but probably very large population
of such neurons, and (2) only by using an almost infinite number of test items would it be possible to measure
approximately the actual ratio of selective neurons in the AM
and HF. It appears to be necessary therefore
to content ourselves with knowledge that these selective
neurons exist in these two centres and not to attempt the meaningless task of
evaluation of the populations of neurons concerned.
Acknowledgements--We thank Dr A. Simpson, Showa University, for advice and help with the manuscript
and Ms M. Yamazaki for typing. This work was supported
partly by the Japanese Ministry of Education,
Science and Culture Grants-in-Aid
for Scientific Research 02404023
and by Nissan Science Foundation.
References
- Amaral DG Memory: anatomical organization of candidate
brain regions. In: Plum F, Mountcastle VB, (eds.),
Handbook of physiology, Vol. 5, Bethesda,
MD, American Physiological Society, pp 221-294, 1987.
- Barloshuk LM The functions of taste and olfaction.
In Schneider LH, Cooper
SJ, Halmi KA (eds). The psychology of human eating disorders (Ann
NY Acad Sci vol 575.
New York, The New York Academy of Science,
pp 353-362,1989.
- Caggiula AR. Stability of behavior produced by
electrical stimulation of the rat hypothalamus. Brain Behav
Evol 1969; 2: 343-358.
- Cohen NJ, Squire LR. Preserved learning and retention
of pattern analyzing skill in amnesia: dissociation
of knowing how and knowing that. Science
1980; 210: 207-209.
- Geschwind N. Disconnexion syndromes in animals
and man. Brain 1965;
88: 237-294.
- Gloor P. Amygdala. In Field J (ed), Handbook of
physiology, neurophysiology, Vol. 2, Washington, American
Physiological Society, pp 1395-1420,1960.
- Horel JA, Keating EG, Misantone LJ. Partial Klulver-Bucy
syndrome produced by destroying temporal neocortex or amygdala.
Brain Res 1975; 94: 347-359.
- Isaacson RL. The Limbic System, 2nd edn, New York,
Plenum Press, 1982.
- Iwai E, Yukie M. Amygdalofugal and amygdalopetal
connections with modality-specific visual cortical areas in Macaques
(Macaca fuscata, M. mulatta, and M. fascicularis). J Comp Neurol
1987; 261: 362-387.
- Jones B, Mishkin M. Limbic lesions and the problem
of stimulus-reinforcement associations. Exp Neurol 1972; 36. 362-377.
- Kaada BR. Stimulation and regional ablation of
the amygdaloid complex with reference to functional representations.
In Eleftheriou BE (ed), The neurobiology of the amygdala, New York,
Plenurn, pp 205-281, 1972.
- Kemble ED, Beckman GJ. Vicarious trial and error
following amygdaloid lesions in rats. Neuropsychol 1970; 8: 161-169.
- Kluver H, Bucy PC. Preliminary analysis of functions
of the temporal lobes in monkeys. Arch Neurol Psychiatr 1935; 42:
979-1000.
- MacLean PD. An ongoing analysis of hippocampal
inputs and outputs: microelectrode and neuroanatomical findings
in squirrel monkeys. In Isaacson RL, Pribram KH (eds), The hippocampus.
Vol. 1: Structure and development, New York, Plenum Press, pp 177-211,
197S.
- Miserendino MDJ, Sananes CB, Melia KR, Davis M.
Blocking of acquisition but not expression of conditioned fear-potentiated
startle by NMDA antagonists in the amygdala. Nature 1990; 345: 716-718.
- Mishkin M, Malamut B, Bachevalier J. Memories and
habits: two neural systems. In Lynch, G, McGaugh, JL, Weinberger
NM (eds), Neurobiology of learning and memory, New York, Guilford,
pp 65-77, 1984
- Nishijo H, Ono T, Nakamura K, Kawabata M, Yamatani
K. Neuron activity in and adjacent to the dorsal arnygdala of monkey
during operant feeding behavior. Brain Res Bull 1986; 17, 847-854.
- Nishijo H, Ono T, Nishino H. Single neuron responses
in amygdala of alert monkey during complex sensory stimulation with
affective significance. J Neurosci 1988; 8: 3570-3583.
- Nishijo H, Ono T, Nishino H. Topographic distribution
of modality-specific amygdala neurons in alert monkey. J Neurosci
1988; 8: 3556-3569.
- Nishijo H, Ono T, Tamura R, Nakamura K. Amygdalar
and hippocarnpal neuron responses related to recognition and memory
in monkey. Prog Brain Res, in press.
- Norgren R, Nishijo H, Travers SP. Taste responses
from the entire gustatory apparatus. In Schneider LH, Cooper SJ,
Halmi KA (eds), The Psychology of Human Disorders (Ann NY Acad Sci
vol 575), New York, The New York Academy of Science, pp 246-264,
1989.
- Ono T, Luiten PGM, Nishijo H, Fukuda M, Nishino
H. Topographic organization of projections from the amygdala to
the hypothalamus of the rat. Neurosci Res 1985; 21: 221-239.
- Ono T, Nishijo H. Neurophysiological basis of the
21 Kluver-Bucy syndrome: Responses of monkey amygdaloid neurons
to biologically significant objects. In Aggleton J (ed), The amygdala:
neurobiological aspects of emotio4 memory, and mental dysfunction
New York, John Wiley & Sons, Inc., in press.
- Ono T, Nishino H, Sasaki K, Fukuda M, Muramoto
K. Role of the lateral hypothalamus and the amygdala in feeding
behavior. Brain Res Bull (Suppl 4) 1980; 5. 143-149.
- Ono T, Nishino H, Sasaki K, Fukuda M, Muramoto
K. Monkey lateral hypothalamic neuron response to sight of food,
and during bar press and ingestion. Neurosci Lett 1981; 21: 99-104.
- Ono T, Nishino H, Nakamura K, Tarnura R, Tabuchi
E. Role of amygdala and hypothalamic neurons in emotion and behaviour
. In Takagi H, Oomura Y, Ito M, Otsuka M (eds), Biowarning system
in the Brain, Tokyo, University of Tokyo Press, pp 309-331, 1988.
- Poletti CE. Is the limbic system a limbic system?
Studies of hippocampal efferents: Their functional and clinical
implications. In Doane KB, Livingstone KE (eds), The limbie system:
functional organization and clinical disorders, New York, Raven
Press, pp 79-94, 1988.
- Poletti CE, Creswell GC. Fornix system efferent
projections in the squirrel monkey: An experimental degeneration
study. J. Comp. Neurol. 175: 101-128, 1977.
- Pribram KH, Bagshaw M. Further analysis of the
temporal lobe syndrome utilizing frontotemporal ablations. J Comp
Neurol 1953; 99: 347-357.
- Rawlins JNP. Associations across time: the hippocampus
as a temporary memory store. Behav Brain Sci 1985; 8: 479-496.
- Russchen FT, Bakst I, Amaral DG, Price JL. The
amygdalostriatal projections in the monkey. An anterograde tracing
study. Brain Res 198S; 329: 241257.
- Schwartzbaum JS. Discrimination behavior after
amygdalectomy in monkeys: visual and somesthetic learning and perceptual
capacity. J Comp Physiol Psychol 1965; 60: 314-319.
- Sclafani A, Belluzzi JD, Grossman SP. Effects of
lesions in the hypothalamus and amygdala on feeding behavior in
the rat. J Comp Physiol Psychol 1970; 72: 394-403.
- Selemon LD, Goldman-Rakic PS. Longitudinal topography
and interdigitation of corticostriatal projections in the rhesus
monkey. J Neurosci 1985; 5: 776-794.
- Spiegler BJ, Mishkin M. Evidence for the sequential
participation of inferior temporal cortex and amygdala in the acquisition
of stimulus-reward associations. Behav. Brain Res 1981; 3: 307-317.
- Squire LR. The hippocampus and the neuropsychology
of memory. In Seifert W (ed), Neurobiology of the hippocampus, London,
Academic Press, pp 491-511, 1983.
- Tamura R, Ono T, Fukuda M, Nishijo H. Role of monkey
hippocampus in recognition of food and nonfood. Brain Res Bull l991;
27: 457-461.
- Zola-Morgan S, Squire LR. Preserved learning in
monkeys with medial temporal lesions: Sparing of motor and cognitive
skills. J Neurosci 1984; 4: 1072-1085.
- Zola-Morgan S, Squire LR, Mishkin M. The neuroanatomy
of amnesia. Amygdala-hippocampus versus temporal stem. Science 1982;
218: 1337-1339.
Copyright © 1996 [Asia Pacific Journal of Clinical
Nutrition]. All rights reserved.
Revised:
January 19, 1999
.
to the top